Bacterial Genomic DNA: Insights into Structure and Function
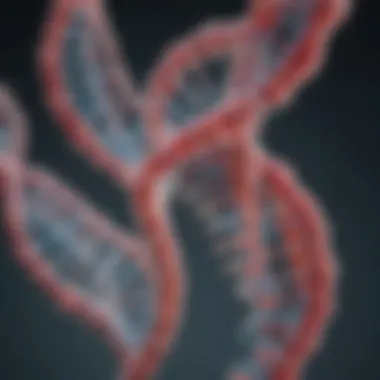
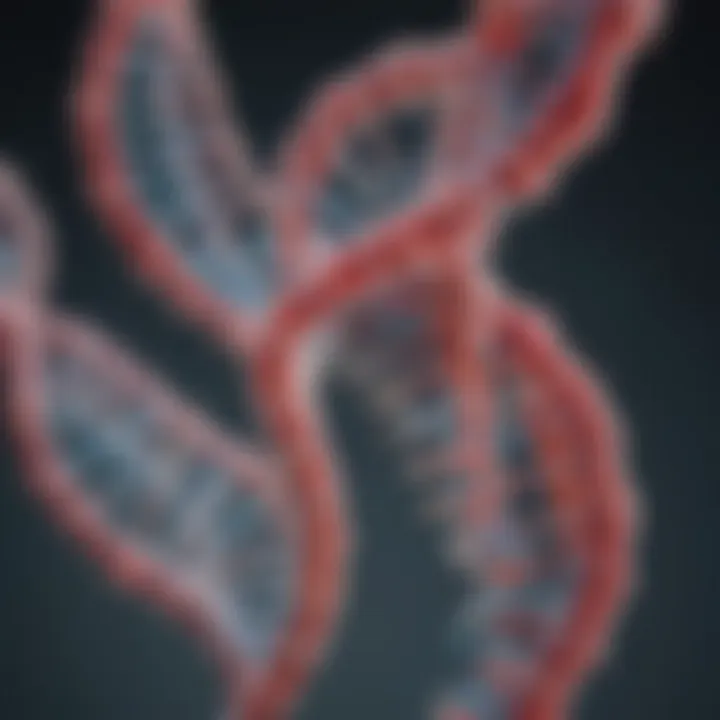
Intro
Bacterial genomic DNA is a fascinating subject that truly deserves a spotlight in contemporary scientific discourse. Unlike the more complex genomes found in eukaryotic organisms, bacterial DNA often exhibits a simplicity that belies its remarkable functions. This article will dive headfirst into the structural oddities of bacterial DNA, the functional roles it plays, and how its study has far-reaching implications in various fields, from medicine to biotechnology.
As we embark on this exploration, we will take a closer look at key aspects such as mechanisms of replication and repair, the impact of genomic variations, and how advancements in genomic studies can translate into tangible applications. Each of these topics interplays intricately with our understanding of microbiology, genetics, and public health, transforming the ways we approach bacterial infections, biotechnology innovations, and genetic research.
Whether you are a student grappling with the basics of microbiology, a researcher delving into genetic elements, or a professional aiming to stay updated on the latest trends in genomic science, this narrative will serve as your comprehensive guide.
Research Overview
Summary of key findings
Throughout our examination, we will encounter several critical findings:
- Structure of Bacterial DNA: Most bacteria possess a single, circular chromosome, which is housed in a region called the nucleoid. This contrasts sharply with eukaryotic organisms, where DNA is linear and compartmentalized within a nucleus.
- Functional Roles: Bacterial DNA is crucial for replication, transcription, and translation — the bedrock processes of gene expression. Bacterial genomes are also replete with mobile elements like plasmids, which can confer advantageous traits, such as antibiotic resistance.
- Genomic Variations: These variations stem from mutations, horizontal gene transfer, and environmental adaptations, contributing to the high level of diversity observed among bacterial species.
Importance of the research in its respective field
The exploration of bacterial genomic DNA is not just an academic exercise; it bears significant implications for a range of applications:
- Antibiotic Resistance: Understanding the genetic mechanisms behind antibiotic resistance is crucial in combating the rising tide of resistant bacteria.
- Biotechnology: Bacterial DNA can be harnessed for biotechnological applications, such as genetic engineering and bioremediation.
- Medical Advances: Insights into bacterial genomes can improve diagnostic methods and vaccine development, ultimately saving lives.
This research not only bolsters our foundational knowledge of bacteria but also equips us with tools to tackle pressing health and environmental issues.
Methodology
Description of the experimental or analytical methods used
In examining bacterial genomic DNA, researchers typically employ a variety of analytical techniques, including:
- Next-Generation Sequencing (NGS): This allows for comprehensive sequencing of bacterial genomes and is pivotal in identifying genetic variations.
- Microarray Analysis: Useful for studying gene expression patterns across different environmental conditions or between bacterial strains.
- Polymerase Chain Reaction (PCR): A common technique that enables amplification of specific DNA segments, facilitating detailed study of genetic sequences.
Sampling criteria and data collection techniques
Creating an accurate picture of bacterial genomes necessitates careful sampling. Scientists often:
- Select bacterial strains representative of diverse environments, from the human gut to extreme environments.
- Utilize genomic databases and repositories to draw comparisons against existing sequences, bolstering the research findings with robust data.
This methodology is geared to foster an in-depth understanding of bacterial genomic DNA, paving the way for innovative solutions to modern scientific challenges.
"The study of bacterial genomes holds the key to an expanding frontier of knowledge in microbiology and genetics, with consequences that could reshape public health and biotechnology."
In sum, unraveling the complexities of bacterial genomic DNA not only enhances our scientific knowledge but also reveals the interconnectedness of biology, health, and technology.
Prologue to Bacterial Genomic DNA
Bacterial genomic DNA is a cornerstone of microbial genetics, acting as the blueprint for life in bacterial cells. Understanding its structure and function can not only illuminate fundamental biological processes but also lead to significant advancements in various fields. This introduction aims to underscore why bacterial genomic DNA is more than just a topic for academic debate—it is the heart of microbiology with implications that reach into biotechnology, medicine, and beyond.
Defining Genomic DNA
At its core, genomic DNA refers to the complete set of genetic information possessed by an organism, stored in the form of DNA sequences. In bacteria, this typically comprises a single circular chromosome along with small, independently replicating DNA molecules known as plasmids. While eukaryotic organisms have a more complex genome organization, bacterial DNA provides a fascinating contrast.
Bacterial genomes tend to be compact, often containing gene-rich regions that allow for efficient cellular activity and rapid adaptation. For example, the genome of Escherichia coli is roughly four to five million base pairs long and harbors about four thousand to five thousand genes. Such a streamlined organization indicates that every nucleotide plays a crucial role, making the study of genomic DNA particularly significant in understanding bacterial functionality and evolution.
Importance in Microbiology
The importance of bacterial genomic DNA extends far beyond its structure. As a focal point in microbiology, understanding this genetic material offers vital insights into bacterial behavior, evolutionary pathways, and ecological roles. By examining how bacteria respond to environmental stresses, researchers can delve into their adaptability and resilience.
"The unique evolutionary strategies employed by bacteria often occur at a pace that's hard to fathom, leading to significant implications in public health and ecology."
One major aspect lies in bacterial adaptability through mechanisms like horizontal gene transfer, allowing for the swift acquisition of new traits, such as antibiotic resistance. This phenomenon not only complicates treatment options but also highlights the importance of genomic studies in developing new therapeutic strategies.
Furthermore, bacterial genomic DNA analysis aids in biosystematics, helping classify and identify bacteria, which can have profound implications in environmental science, agriculture, and medicine. As industry and research increasingly leverage genomic information, the potential applications become more apparent, paving the way for innovations in fields like vaccine development, disease diagnosis, and bioengineering.
Ultimately, a comprehensive knowledge of bacterial genomic DNA is essential for those involved in microbiological research and applications. It lays the groundwork for tackling pressing global challenges, ranging from infectious diseases to food security and environmental sustainability.
Structural Characteristics of Bacterial Genomic DNA
Understanding the structural characteristics of bacterial genomic DNA is crucial for several reasons. Firstly, the structure directly influences the function of the DNA—it determines how genes are expressed, how they are replicated, and how they can adapt to changes in the environment. The specific arrangement of the DNA, including the chromosomal structure and the presence of plasmids, plays an integral role in bacterial physiology and survival strategies.
In this section, we will dissect the fundamental elements of bacterial genomic DNA, which encompass chromosomal structures and the roles of plasmids.Whether we look at a common bacterium like Escherichia coli or more complex organisms, the insights derived from the structural overview can resonate across diverse fields, including microbiology, genetic engineering, and biotechnology.
Chromosomal Structure
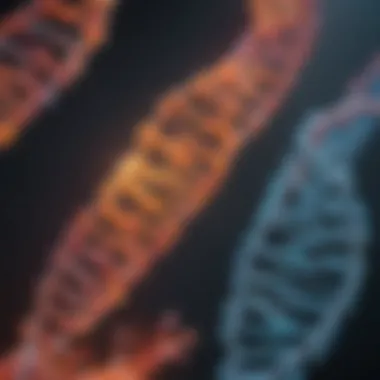
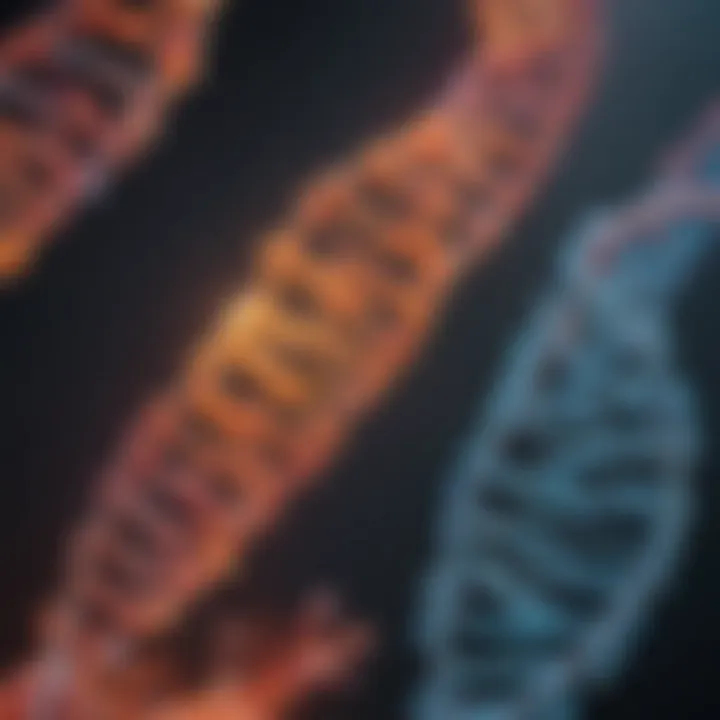
The chromosomal structure of bacterial genomic DNA is notably distinct compared to eukaryotic organisms. Bacteria typically possess a single, circular chromosome located in a region called the nucleoid. This unique configuration is not merely a twist of fate; it’s an evolutionary adaptation that allows for more efficient replication and transcription processes.
- Nucleoid Region: The absence of a membrane-bound nucleus means that the chromosomal DNA is freely located in the cell cytoplasm. This proximity facilitates a rapid response to environmental changes. The chromosomal DNA is compacted through supercoiling, which helps fit the entire length of DNA within the confined bacterial cell.
- Gene Clusters: Batch files of genes known as operons are often found along the chromosome, providing an organized approach to gene expression. This strategic placement enables bacteria to optimally utilize resources and energy under varying conditions.
The significance of studying the chromosomal structure lies not just in its biology but in its applications. Insights into the chromosomal architecture can guide genetic engineering efforts, biotechnological applications, and antibiotic target development. Understanding which elements are crucial within the chromosomal structure can open doors to innovations in microbial treatment and manipulation.
Plasmids and Their Functions
Plasmids are small, circular pieces of DNA that exist independently of the chromosomal DNA within bacterial cells. They serve several essential functions and have become significant players in the landscape of bacterial genetics.
- Antibiotic Resistance: One of the most notable roles of plasmids is their capacity to carry genes that confer antibiotic resistance. This ability allows bacteria to survive in hostile environments where antimicrobial agents are present. The spread of these resistance traits among bacterial populations contributes to the pressing issue of antibiotic resistance.
- Genetic Engineering: Plasmids are also pivotal in the realm of biotechnology. Scientists harness plasmids as vectors to introduce foreign genes into bacteria, aiming to produce proteins of interest or create genetically modified organisms. Their ease of manipulation and replication makes them invaluable tools in molecular biology labs.
"Plasmids are a two-edged sword; they can contribute to both the advancement and the risk within microbial management."
- Metabolic Functions: Beyond these capabilities, some plasmids carry genes that enhance metabolic functions, like those that enable bacteria to degrade environmental pollutants. This ability is particularly important in bioremediation efforts, targeting contaminated sites and mitigating ecological hazards.
In summary, the structural characteristics of bacterial genomic DNA illuminate the intricacies of bacterial life. The interplay between chromosomal organization and plasmid function underpins much of what we know about bacterial behavior and adaptability. As we delve into subsequent sections of this article, these foundational elements will serve as a backdrop to the exploration of replication mechanisms, DNA repair, and the dynamic nature of bacterial genomes.
Genomic Organization
Genomic organization in bacteria fundamentally shapes not just their survival mechanisms but also influences their adaptability and evolution. Understanding how bacterial genomes are structured offers insights into a range of biological processes, including gene regulation, expression, and the overall functionality of the organism. This can have far-reaching implications in microbiology, biotechnology, and even medical research.
Gene Density and Distribution
Gene density refers to the number of genes present in a given length of DNA. In bacterial genomes, gene density is typically high, which might come as a surprise to those accustomed to thinking about eukaryotic genomes, which often contain large amounts of non-coding DNA. A prime example can be observed in Escherichia coli, which boasts about 4300 genes packed within roughly 4.6 million base pairs. This high density enables bacteria to efficiently carry out essential functions and adapt rapidly to changing environments.
The distribution of genes within the genome is not random; rather, it follows distinct patterns. For instance, essential genes often cluster together, enhancing their co-regulation. This arrangement allows bacteria to swiftly respond to environmental pressures—for example, when a specific nutrient is scarce, genes involved in its metabolism may rapidly be expressed in unison. Such genetic organization is crucial for the bacterium's ability to thrive under various conditions, demonstrating the functional significance hidden behind genomic structures.
Furthermore, irregularities in gene density and distribution can hint at evolutionary pressures. Bacteria living in stable environments often exhibit lower variability in their gene distribution, while those with a more dynamic habitat can present a patchwork of gene clusters that reflect their varied lifestyles.
Operons and Regulation
As bacteria maneuver through their environments, they must precisely regulate their gene expression. This is often achieved through the organization of genes into operons. Operons are groups of genes that are transcribed together as a single mRNA molecule, allowing for coordinated regulation. The classic example is the lac operon in E. coli, governing lactose metabolism. When lactose is present, the operon is activated, leading to the expression of genes necessary for its breakdown. When lactose is absent, the operon is silenced, conserving energy and resources.
The regulatory mechanisms controlling operons can be intricate, involving proteins that bind to specific sequences of DNA. This can either enhance or inhibit transcription, allowing bacteria to finely tune their responses to environmental signals.
The beauty of operons lies not just in their efficiency but in their elegance. By grouping functionally related genes, bacteria can turn on or off entire pathways as a single unit, embodying a sophisticated orchestration of genetic regulation.
Moreover, the role of regulatory elements, such as promoters and operators, cannot be overlooked. These components determine when and how actively an operon is expressed, directly impacting the bacterium's adaptability to shifting environmental conditions.
In summary, the genomic organization in bacteria, encompassing gene density and the arrangement of operons, highlights its essential role in their survival and evolutionary strategy. An understanding of these elements opens doors to significant breakthroughs in fields like biotechnology and medicine, where manipulating these systems could lead to innovative solutions to pressing human challenges.
Check out more on bacterial genome organization here for deeper insights.
Mechanisms of DNA Replication
Understanding the mechanisms of DNA replication is a cornerstone in the study of bacterial genomic DNA. This process is critical for cellular proliferation, ensuring that genetic information is accurately passed on from one generation to the next. When an organism divides, its DNA must duplicate precisely; any error in this endeavor can have profound implications for the viability—or even the survival—of the bacterium. It’s not just a mere copying of the genetic material; rather, it involves intricate pathways and techniques that have evolved over time, bringing us to today’s rich fabric of microbial life.
Key points about replication include how it empowers genetic continuity, facilitates evolutionary adaptation, and serves as a basis for various biotechnological applications. By delving into the specifics of initiation, elongation, and termination, we can grasp the remarkable coordination of enzymes and factors at play, which uphold the fidelity of replication and the stability of the bacterial genome.
Replication Initiation
The initiation of DNA replication is a highly regulated process. It begins at specific locations known as origins of replication, where the double helix unwinds, creating a replication bubble. In bacteria, this usually occurs at a unique site called the oriC region. Initiator proteins bind to this site, recruiting helicase, which proceeds to separate the strands of the DNA, forming two single-stranded templates.
During this stage, several key components come into play:
- DnaA protein that recognizes the oriC, begins unwinding the DNA.
- DnaB, the helicase, that unwinds the DNA helix, allowing the replication machinery access.
- Single-strand binding proteins prevent the re-annealing of DNA strands, stabilizing the unwound region A classic example of a system in action, DnaA also serves as a regulator, showing just how delicate this balance of proteins is.
In summary, replication initiation is not random or haphazard—it’s a precise orchestration that prepares the way for the subsequent steps of replication, setting the stage for efficient DNA duplication.
Elongation and Termination
As replication progresses, the elongation phase begins. Here, DNA polymerase enzymes take the center stage. They add nucleotides to the growing DNA strand in a 5’ to 3’ direction. This step is fraught with complexity; bacterial DNA polymerases must ensure not just speed but also accuracy in pairing nucleotides. The enzyme’s proofreading ability enables it to backtrack and correct errors, maintaining the integrity of the genetic code.
During this phase, the replication fork moves at a breathtaking pace—an impressive 1,000 nucleotides per second in E. coli. The overall efficiency relies heavily on proximity of components:
- Leading strand synthesized continuously, while the lagging strand consists of Okazaki fragments requiring additional coordination.
- Clamp loaders and sliding clamps act like a team of relay runners, ensuring that the DNA polymerase remains tethered to the DNA template, thus optimizing the speed and continuity of DNA synthesis.
Once the DNA polymerase has completed its task and replicated the entire DNA strand, the termination stage comes into play. Specific terminator sequences signal the end of replication, and new strands are separated from their templates. Here, other enzymes, including DNA ligases, stitch together any gaps left on the lagging strand, ensuring a smooth finish. This is where precise regulation of enzyme activity is crucial; missteps can result in incomplete genomes.
"Replication is a dance of enzymes, each moving in perfect sync to ensure that the genetic legacy of the bacterium lives on."
Overall, unraveling the mechanisms behind DNA replication is not just an academic exercise; it is vital for various applications including genetic engineering and developing antibiotics. Each step, from initiation to termination, showcases how life has optimized replicative integrity across millions of years, a testament to the intricate world of microbology.
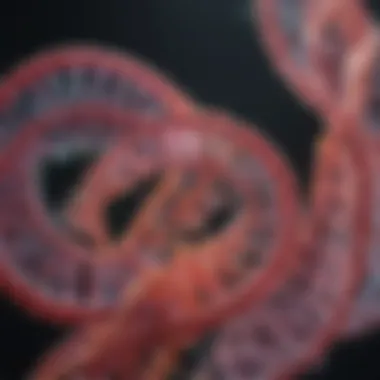
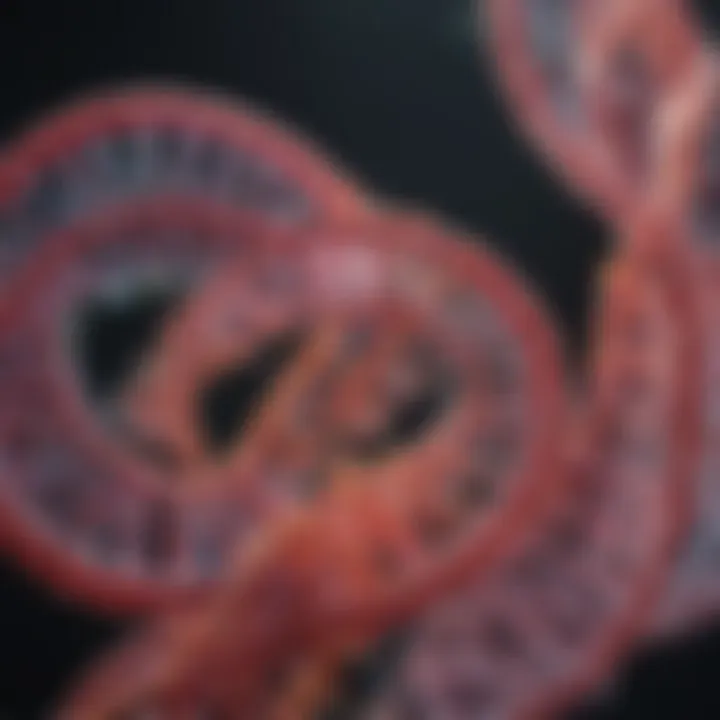
DNA Repair Mechanisms
The integrity of bacterial genomic DNA is critical for the survival and proliferation of these microorganisms. When damaged, the consequences can range from loss of function to cell death. Understanding DNA repair mechanisms is essential not only for grasping how bacteria maintain their genomic stability but also for appreciating the implications in fields like biotechnology and medicine. Insight into these processes unveils intricate biological responses that highlight bacteria's resilience and adaptability amidst environmental challenges.
Types of DNA Damage
Bacterial DNA can sustain various forms of damage, each necessitating specific repair mechanisms. Here are some categories of DNA damage encountered:
- Physical Damage: This includes breaks in the DNA strand or distortions caused by radiation, such as UV light. Such damage often leads to what is known as double-strand breaks, which can be lethal if not appropriately repaired.
- Chemical Damage: Exposure to reactive oxygen species or certain chemicals can result in base modifications or strand scissions. For example, alkylation, a process where alkyl groups are added to DNA, can hinder replication and transcription processes.
- Replication Errors: During DNA replication, errors can occur, leading to mismatches or incorporation of erroneous bases. This not only affects gene expression but can also lead to mutations that may become permanent if not rectified.
- Interstrand Crosslinks: These occur when both strands of the DNA helix are covalently bonded together, preventing the separation necessary for replication and transcription. Such crosslinks are particularly hazardous as they impinge on essential cellular functions.
"DNA repair is not merely a backup system; it’s an active participant in the life cycle of bacteria, functioning as both shield and sword against myriad threats to genomic stability."
Repair Pathways
Bacteria employ a myriad of repair pathways, each tailored to address distinct types of DNA damage. The principal pathways include:
- Mismatch Repair (MMR): This pathway focuses on correcting mismatched bases that can arise during DNA replication. It increases the fidelity of genetic information transfer.
- Base Excision Repair (BER): Dealing specifically with small, non-helix-distorting base lesions, BER involves the removal and replacement of damaged bases to maintain DNA integrity.
- Nucleotide Excision Repair (NER): This mechanism operates by excising large, helix-distorting DNA lesions, such as those caused by ultraviolet light, and restoring the strand’s continuity.
- Homologous Recombination (HR): When faced with double-strand breaks, bacteria can utilize HR as a repair mechanism by using a homologous sequence as a template for repair, ensuring accurate restoration of the damaged region.
- Non-homologous End Joining (NHEJ): In cases where homologous regions are not available, bacteria may resort to NHEJ, directly ligating the broken ends to re-establish DNA continuity, though this method does carry a higher risk of introducing mutations.
- SOS Response: Under severe stress, a more generalized repair system known as the SOS response is activated. This system allows bacteria to handle extensive DNA damage but often leads to increased mutation rates, a double-edged sword in the evolution of bacteria.
Through these pathways, bacteria not only address immediate threats to their genomic integrity but also adapt to challenging environments, lending insight into their evolutionary strategies and potential applications in genetic engineering and therapeutics.
Genomic Variability in Bacteria
Genomic variability in bacteria serves as a cornerstone for understanding the evolution and adaptability of these organisms. This variability allows bacteria to thrive in diverse and often challenging environments. It's critical because it can have profound implications for their survival, pathogenicity, and interaction with humans and other organisms. Grasping this variability is key to advances in fields such as microbiology, medicine, and biotechnology.
Mutations and Adaptations
Mutations are the genetic changes that occur in an organism's DNA. In bacteria, mutations can happen for several reasons: errors during DNA replication, exposure to mutagenic agents, or even as a response to environmental stress. These mutations can be categorized as either neutral, detrimental, or beneficial.
- Neutral mutations do not affect the organism’s fitness and can be passed on through generations.
- Detrimental mutations may hinder the bacteria's ability to survive or reproduce, often leading to a die-off.
- Beneficial mutations, however, enhance survival. For instance, a bacterium might mutate to develop resistance against an antibiotic, effectively improving its survival odds in a hostile environment.
This constant shuffle of genetic material allows bacteria to adapt quickly. For example, consider the case of Escherichia coli – known for its rapid growth and adaptability. Some strains have developed resistance to multiple types of antibiotics, making treatment increasingly difficult.
"A single mutation can make the difference between a benign strain and a deadly pathogen."
This adaptability is not merely a random process; it’s often driven by environmental pressures. Bacteria living in nutrient-scarce environments are more likely to undergo beneficial mutations that improve their nutrient uptake.
Horizontal Gene Transfer
Horizontal gene transfer (HGT) is another way bacteria acquire genetic material, allowing genes to move across species lines. It contrasts sharply with vertical gene transfer, where genetic material is passed from parent to offspring. HGT can occur through various mechanisms, including transformation, transduction, and conjugation.
- Transformation involves the uptake of free DNA from the environment, which can come from dead bacterial cells.
- Transduction happens when bacteriophages (viruses that infect bacteria) introduce new DNA into a cell.
- Conjugation is the direct transfer of DNA from one bacterial cell to another through specialized structures called pili.
The significance of horizontal gene transfer is profound. For instance, it explains how a non-pathogenic bacterium can quickly obtain drug resistance traits from a pathogenic strain, thereby becoming a threat to public health. Bacteria can share genes that confer advantages such as antibiotic resistance, increased virulence, or enhanced metabolic pathways, giving rise to new strains that may be better adapted to their environments.
This concept of sharing genetic information contributes to the swift evolution of bacterial communities, often leading to challenges in treating infections. With the rapid change in the genetic landscape, medical interventions face a moving target, making research into HGT crucial for future therapeutic approaches.
Applications of Bacterial Genomic Studies
The study of bacterial genomic DNA has broadened the horizons of both science and industry, bringing forth an array of applications that benefit sectors ranging from healthcare to environmental management. Understanding genomic structures can lead to remarkable breakthroughs. In this section, we will dive into two primary applications: biotechnology innovations and medical implications. These areas capture the essence of why bacterial genomics holds a pivotal place in contemporary scientific endeavors.
Biotechnology Innovations
Bacterial genomics serves as a bedrock for biotechnological advancements. The contributions to fields such as agriculture, biofuels, and synthetic biology are noteworthy. One clear example is the development of genetically modified organisms (GMOs), where bacterial genes are incorporated into plants to enhance resistance against pests. This demonstrates how genomic studies enable the engineering of traits that improve crop yields and sustainability.
Moreover, bacteria play a vital role in the production of biofuels. By utilizing metabolic engineering, scientists can manipulate bacterial pathways to convert biomass to fuel more efficiently. Enzymes encoded by bacterial DNAs are harnessed to break down complex organic materials, which is a game changer for renewable energy sources.
- Key benefits of leveraging bacterial genomics in biotechnology include:
- Enhanced agricultural productivity through GMOs.
- Sustainable energy production via biofuel manufacturing.
- Rapid development of bioprocesses for producing valuable compounds.
This emphasis on innovation highlights a critical synergy between understanding bacterial genomes and applying that knowledge to real-world challenges. With the help of genomic data, researchers can prioritize traits for development, potentially yielding crops that can thrive in changing environmental conditions.
Medical Implications
On the medical front, bacterial genomic studies are a treasure trove leading to the development of novel diagnostics and therapeutics. The advent of next-generation sequencing technologies has revolutionized how we view pathogens. Rapid identification enables healthcare professionals to determine the specific genome of a pathogen, allowing for tailored treatments that are more effective.
For instance, understanding the resistance mechanisms of bacteria through genomic studies aids in pinpointing why certain antibiotics may fail. This sparks the creation of new antibiotics designed to target resistant strains, enhancing our ability to combat antimicrobial resistance.
In addition to therapeutics, genomic insights can significantly improve public health initiatives:
- Infectious disease tracking: Genomic data allows for better monitoring of disease outbreaks, as scientists can trace mutations and spread patterns of pathogens.
- Personalized medicine: By analyzing individual genomes, treatments can be customized to suit specific genetic makeups.
"The intersection of genomics and health is critical. We are standing on the edge of a major leap in how we approach treatment and prevention of diseases."
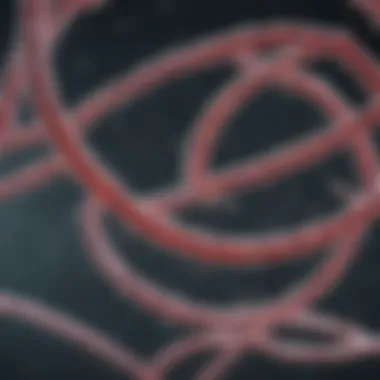
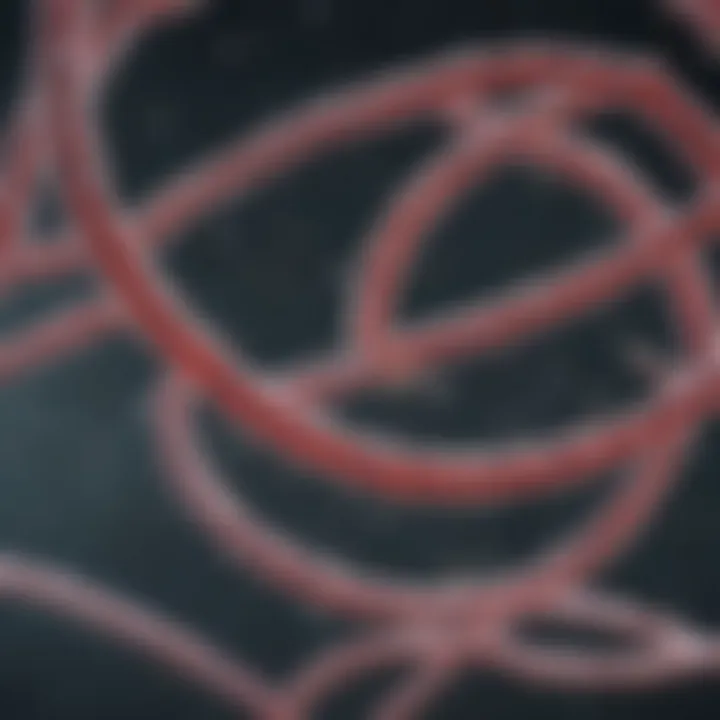
The implications of genomic research on public health cannot be overstated. With increased understanding comes the power to control outbreaks and enhance patient outcomes. This interplay between biology, technology, and medicine fosters an environment where efficiency and effectiveness become the norm.
In summary, the applications of bacterial genomic studies are not merely theoretical; they hold practical, transformative potential. From innovating biotechnological processes to revolutionizing medical approaches, the benefits of comprehensively understanding bacterial genomes are vast and indispensable.
The Role of Bioinformatics
Bioinformatics serves as a bridge between biological data and computational analyses, playing an indispensable role in the study of bacterial genomic DNA. Traditional methods of examining genetic material have grown increasingly outdated and cumbersome, whereas bioinformatics empowers researchers to tackle vast datasets with ease and precision. In bacterial genomics, this translates to enhanced understanding of the genetic underpinnings that dictate microbial behavior, adaptability, and evolution. The applications of bioinformatics are broad, affecting everything from identifying novel genes to understanding the complex interactions in microbial ecosystems.
Allowing for the analysis of massive genomic datasets, bioinformatics equips scientists with the necessary tools to discern patterns, structures, and relationships within and between genomes. The growth in sequencing technology provides an overwhelming amount of data, and without proper analytic frameworks, this information can be daunting to manage. Through sophisticated algorithms and data-mining techniques, bioinformatics not only organizes this information but also extracts significant biological insights, shaping our understanding of bacterial behavior and its implications in fields such as medicine, agriculture, and environmental science.
Analyzing Genomic Data
The process of analyzing genomic data in bacterial studies begins with the acquisition of sequence data from various sources, such as whole-genome sequencing projects or targeted studies. For instance, when researchers sequence the genetic material of Escherichia coli, they may gather information from different strains existing in varying environments. This data must then undergo quality checks and filtering to ensure its reliability. After culling low-quality sequences, scientists employ bioinformatics tools such as BLAST (Basic Local Alignment Search Tool) to compare sequences against a database, discovering homologies and variations among different bacteria.
Moreover, the analysis often involves annotation of the genomes, where gene prediction software identifies the locations of genes and assigns functions based on existing knowledge. Techniques like RNA-Seq allow for the evaluation of gene expression profiles, revealing insights into how bacterial populations respond to environmental stimuli. This detailed analysis of genomic data sheds light on the evolutionary relationships and metabolic capabilities of bacteria, paving the way for novel biotechnological applications.
Comparative Genomics
Comparative genomics takes the principles of genomic analysis a step further by enabling researchers to scrutinize the genomes of different bacterial species side-by-side. This technique provides invaluable insights into the evolution of microbes, revealing genetic variations that may be responsible for distinct traits between species or strains. By comparing the genome of a pathogenic bacterium such as Staphylococcus aureus with that of a non-pathogenic relative, researchers can identify specific genes or genomic regions that confer virulence, antibiotic resistance, or other critical phenotypes.
Utilizing bioinformatics, comparative genomics also facilitates the identification of conserved and divergent regions across genomes. This not only enhances our understanding of the fundamental biology of bacteria but also assists in pinpointing essential genes for potential therapeutic targets. Furthermore, this approach may highlight regions of genomic plasticity, which are hotspots for horizontal gene transfer events, emphasizing the evolutionary dynamism of bacterial populations.
In summary, bioinformatics emerges as an essential discipline that transforms the vast and complex data surrounding bacterial genomic DNA into coherent studies. By applying methodologies in analyzing genomic data and engaging in comparative genomics, scientists can unlock the secrets of bacterial life, leading to significant advancements in health, industry, and environmental management.
"Job of bioinformatics is not just to crunch numbers; it's about telling a story through data and enabling discoveries that could save lives."
Bioinformatics thus stands as a key driving force for innovation and progress in bacterial genomic research.
Ethical Considerations in Genomic Research
In the realm of bacterial genomics, ethical considerations hold immense significance. With advancements in genetic engineering and genomic studies, we face a plethora of moral questions that can't simply be brushed aside. These issues can affect not only scientific integrity but also public trust in research and its applications in healthcare and biotechnology.
Considering the benefits of genomic research, it is crucial to weigh these against potential risks. On the one hand, insights gained from bacterial genomic studies can lead to innovative treatments and the development of new technologies. On the other hand, the manipulation of genetic material brings up debates surrounding safety, consent, and accessibility.
Bioethics in Genetic Engineering
Digging deeper into bioethics within genetic engineering, we find a mine of complexities. The modifications made to bacterial genomes can pave the way for significant breakthroughs. For instance, genetically modified bacteria can be engineered to synthesize insulin or produce biofuels, revolutionizing sectors such as medicine and energy. However, who decides what's acceptable? The ethical frameworks guiding these decisions often seem nebulous.
The principles of bioethics often include respect for autonomy, beneficence, non-maleficence, and justice. These come into play when determining how research is conducted and who benefits from the outcomes. Worries about 'designer bacteria' proliferating without sufficient oversight have made ethical considerations even more pressing. When we start manipulating living organisms, the questions multiply:
- Is it right to alter a bacterium's genome?
- What are the long-term impacts on ecosystems?
- How do we ensure that the benefits of such technologies reach those in need, rather than just big corporations?
These questions underscore the necessity of robust guidelines in genetic engineering, ensuring that scientific endeavor does not come at an unacceptable ethical cost.
Public Health Implications
A significant aspect of genomic research intersects clearly with public health implications. The last few years have highlighted just how vital our understanding of bacterial genomics can be in tackling pathogens, especially during global health crises.
When considering public health, there's the layer of responsibility researchers must acknowledge surrounding their findings. Genomic advancements can lead to vaccines, antibiotics, and diagnostic tools that save countless lives. Yet, the release and application of these technologies must be approached with caution.
"Scientific progress should not outpace ethical reflection; we shouldn't leave humanity behind while racing toward innovation."
Here are some considerations:
- Access to Technologies: Who benefits from these advancements?
- Risk of Misuse: How do we regulate knowledge transfer when it could be used for harmful purposes?
- Cultural Sensitivity: Are different communities' values respected in genomic studies?
With these aspects weighing heavily, the conversation on ethics and public health becomes not just a matter of theory, but one of practical reality. In an interconnected world, the repercussions of genomic research can extend far beyond the lab, affecting communities, healthcare policies, and even global justice.
Future Directions in Bacterial Genomics
The field of bacterial genomics is advancing at a breakneck speed, propelled by technological innovations and an increasing understanding of the microbial world. This segment delves into why exploring future directions in bacterial genomics is crucial, highlighting areas that can lead to significant breakthroughs—from new methodologies to better understanding of microbial ecosystems. The implications of these developments extend far beyond academia, permeating industries like healthcare, agriculture, and environmental management.
Emerging Technologies
A multitude of novel technologies is beginning to reshape how researchers approach bacterial genomics. High-throughput sequencing, for example, has dramatically changed the landscape. It allows for rapid sequencing of entire genomes, making it feasible to analyze complex microbial communities in their environmental contexts.
- Single-Cell Sequencing: This technique opens up new horizons by enabling scientists to study individual bacteria rather than averages from a population. Notably, it helps in understanding the role of rare species within a community that could otherwise remain hidden.
- CRISPR-Cas9: This revolutionary gene-editing tool isn't just for eukaryotes; it also has significant applications in bacteria. By pinpointing and editing bacterial genomes with precision, researchers can explore gene functions in ways previously thought impossible.
- Machine Learning and AI: The use of artificial intelligence in genomic research allows for more sophisticated data analyses. Algorithms can detect patterns and predict functionalities based on genetic sequences that a human might overlook, streamlining research significantly.
These advancements present numerous advantages, from faster data acquisition to more insightful interpretations of genomic data.
Potential Research Avenues
Emerging technologies pave the way for diverse research avenues in bacterial genomics. Understanding these new pathways can lead to groundbreaking discoveries.
- Microbiome Studies: There is an increasing focus on the human microbiome and its influence on health. Investigating how various bacterial species interact within their environment could uncover vital links to diseases such as obesity, diabetes, and inflammatory conditions.
- Synthetic Biology: Tailoring bacterial genomes to produce desired compounds has tremendous potential. For instance, designing strains that can convert agricultural waste into biofuels or creating bacteria that synthesize pharmaceuticals could address significant industrial challenges.
- Environmental Remediation: By tweaking the genetic makeup of certain bacteria, researchers could enhance their abilities to break down pollutants or absorb heavy metals. This approach may eliminate toxins from soil and water, leading to sustainable environmental practices.
- Antibiotic Resistance Research: With bacterial resistance on the rise, exploring genomic factors that contribute to this phenomenon is crucial. Identifying specific genes linked to resistance can inform the development of new antibiotics or treatment strategies.
In closing, the journey of bacterial genomics stands at a crossroads of innovation and necessity. As research ventures into these potential avenues, the future holds a promise for not only advancing scientific understanding but also addressing some of society's most pressing challenges.
"The future of healthcare, agriculture, and environmental solutions will depend heavily on our understanding of bacterial genomes and the innovative approaches we take to manipulate them."
Thus, as efforts grow to bridge the gap between skills and discoveries, the road ahead looks promising.