Exploring the DNA Model: Structure and Significance
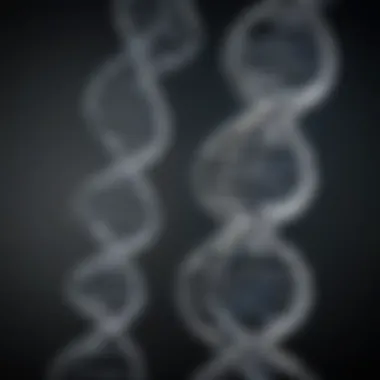
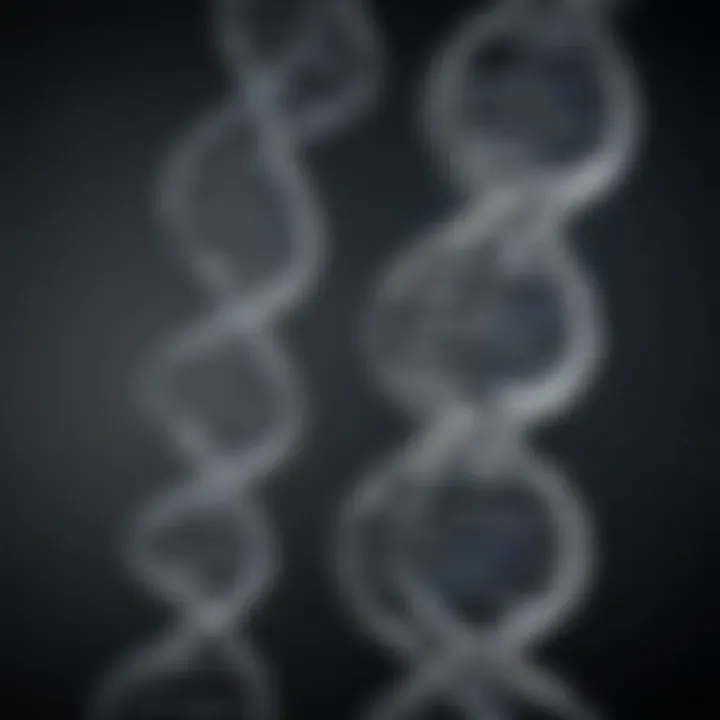
Intro
The exploration of the DNA model marks a pivotal turning point in biological sciences, shaping our understanding of life itself. Many scientists have undertaken profound journeys to peel back the layers of genetics, revealing how this intricate molecule operates as the blueprint of life. Delving into the structure of DNA isn't simply a task for the laboratory; it's an invitation to witness the interplay between history, biology, and technology.
Research Overview
Summary of Key Findings
The current discourse around DNA emphasizes its complex architecture and function. From the discovery of its double helix structure by James Watson and Francis Crick in 1953 to modern advancements such as CRISPR, the findings illuminate several critical facets:
- Nucleotide Composition: DNA consists of nucleotides, which are the building blocks. Each nucleotide is made up of a phosphate group, a sugar molecule, and a nitrogenous base. The sequence of these bases encodes genetic information.
- Double Helix Formation: The unique double helix structure allows DNA to compactly store immense genetic information while facilitating replication.
- Base Pairing Mechanism: Adenine pairs with thymine, and cytosine pairs with guanine. This pairing is essential for the accurate transmission of genetic information during cell division.
- Gene Expression Role: Genes are segments of DNA that, when expressed, lead to the production of proteins. This process is crucial for various functions within an organism.
Importance of the Research in Its Respective Field
Understanding the DNA model is foundational not just for biology but also for various interdisciplinary applications. It serves as a cornerstone for genetics, medicine, agriculture and forensic science, among others. Advances in DNA research have fundamental implications:
- In medicine, genetic mapping enhances disease understanding and treatment options.
- In agriculture, genetic modifications improve food security and resilience against pests.
- In forensics, DNA profiling helps solve crimes and exonerate the innocent.
All these applications underscore the necessity of comprehending DNA’s structure and functionality as they continually influence scientific and societal advancements.
Methodology
Description of the Experimental or Analytical Methods Used
The methodologies surrounding DNA research are varied and complex. Key techniques employed include:
- Polymerase Chain Reaction (PCR): This method amplifies small segments of DNA, making it possible to analyze even minute samples.
- Gel Electrophoresis: After PCR, gel electrophoresis separates DNA fragments based on size, allowing for visualization and analysis.
- DNA Sequencing: This is critical for understanding the sequence of bases. Techniques like Sanger sequencing and next-generation sequencing have revolutionized our ability to explore genomes.
Sampling Criteria and Data Collection Techniques
When examining specific DNA samples, diverse criteria come into play:
- Source: Samples can be extracted from blood, saliva, or even hair follicles, depending on the research question.
- Quality Control: Ensure that samples are uncontaminated and viable for analysis, which often involves stringent storage conditions and handling protocols.
- Ethical Considerations: Researchers navigate consent issues, particularly when human samples are used, ensuring that all protocols adhere to ethical guidelines.
This methodological framework allows scientists to construct a robust understanding of DNA, leading to insights that resonate through multiple fields of study.
Foreword to DNA
Understanding DNA is akin to peering into nature's blueprint. At the core of biological sciences, DNA is not just a molecule; it's the key to life itself. In this section, we aim to unravel the layers of DNA—from its historical significance to its critical role in shaping biology as we know it today. By exploring DNA, we glean insights into the very mechanisms of heredity and the foundational processes that govern life.
Historical Overview
The history of DNA is a rich tapestry interwoven with various scientific discoveries. In the mid-19th century, a Swiss physician named Friedrich Miescher was the first to isolate nucleic acids from cell nuclei, though he didn't yet know what he had. Later, in the early 20th century, scientists like Phoebus Levene started to identify the components of DNA, leading to the discovery of nucleotides—those tiny building blocks of life.
But it was not until 1953 that James Watson and Francis Crick proposed the double helix structure that we are familiar with today. With the base pairing of adenine to thymine and guanine to cytosine, their model not only explained how genetic information is stored but also provided a framework for understanding replication. The famous publication in Nature set the stage for genetics to blossom as a science.
Importance of DNA in Biology
From a biological perspective, DNA is invaluable. It serves as the repository for genetic information, instructing the formation of proteins essential for cellular functions. This genetic code is what differentiates one organism from another, underpinning diversity in the biosphere. Moreover, DNA's role in heredity, where it passes traits from one generation to the next, fosters evolutionary changes over time. Any conversation about biology inherently circles back to DNA, underscoring its profound relevance across various fields, whether in medicine, agriculture, or conservation.
"DNA is like a software program but far, far more complex than anything we’ve ever created."
In summary, the significance of DNA cannot be overstated. Its historical journey demonstrates centuries of discovery, while its biological importance highlights its foundational role in life on Earth. Understanding DNA is vital not just for scientists, but for anyone interested in the intricate workings of life itself.
Molecular Structure of DNA
In the domain of biological sciences, understanding the molecular structure of DNA is like unveiling a treasure map that leads to the mysteries of life. It's not just about knowing the components; it's about how they interact and form the basis of genetic information. This structure is foundational, influencing everything from heredity to the intricacies of life itself.
Components of DNA
Nucleotides
Nucleotides are the building blocks of DNA. They consist of three components: a phosphate group, a five-carbon sugar, and a nitrogenous base. The unique characteristic of nucleotides is their ability to form long chains through a series of phosphate-sugar linkages while also carrying the essential genetic information through their sequence of bases. This dual role is what makes nucleotides a critical focus within the study of DNA. The order of these bases dictates the genetic instructions through which an organism's traits are expressed, making them a popular choice for genetic research and engineering.
However, one must note the inherent variability in nucleotides as they can be sensitive to environmental factors which may lead to mutations. This aspect presents both challenges and opportunities in the fields of genetics and molecular biology.
Phosphate Backbone
The phosphate backbone provides structural integrity to the DNA molecule, anchoring the nucleotides in place and allowing them to link together in a robust manner. One key trait of the phosphate backbone is its negativity, which helps in the stabilization of the complex through ionic interactions with positively charged proteins. This characteristic satisfies a major requirement for DNA as a storage medium for genetic material.
Moreover, the phosphate groups of DNA play significant roles in cellular processes, such as providing energy through the formation of ATP. On the flip side, the rigidity of this backbone can sometimes inhibit flexibility; however, it ensures that the structural integrity of DNA withstands the mechanical strains of cellular processes.
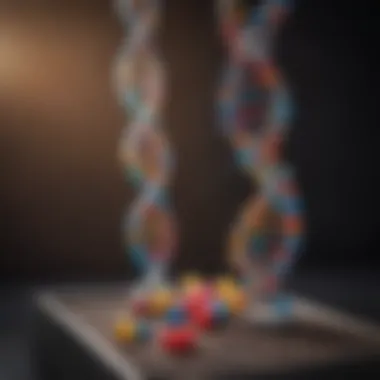
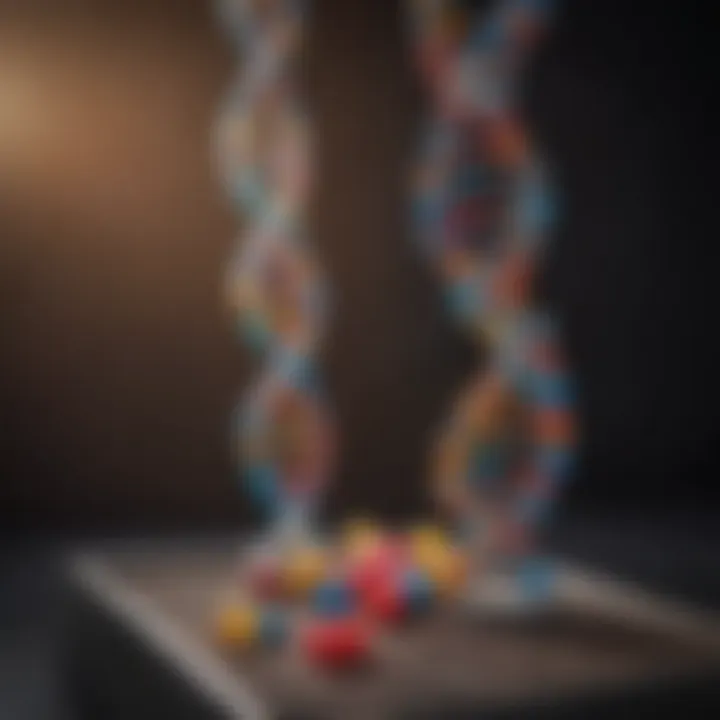
Nitrogenous Bases
The nitrogenous bases—adenine, thymine, guanine, and cytosine—are critical for carrying genetic information. Each base has a specific pairing characteristic: adenine pairs with thymine, while guanine pairs with cytosine. The complementary nature of these bases is fundamental for DNA's ability to replicate and transmit genetic information accurately. This defining feature of nitrogenous bases allows for the formation of hydrogen bonds, enabling the helical structure of DNA to remain stable while also permitting the dynamic processes of transcription and replication.
The unique combination of bases in an organism not only determines genetic diversity but also establishes specific functions and properties, making nitrogenous bases a focal point in genetic studies and applications.
Double Helix Configuration
Discovery by Watson and Crick
The double helix configuration of DNA, famously elucidated by James Watson and Francis Crick in the early 1950s, marked a seminal moment in molecular biology. Their work revealed that two strands of DNA run in opposite directions, twisting around each other to form a helical structure. This characteristic provides stability and integrity to the DNA molecules, making them resilient to environmental factors that might lead to degradation.
Watson and Crick's discovery was instrumental in understanding the foundational principles of genetics. By mapping how genetic information is stored and replicated, their work opened floodgates to numerous advancements in genetic research and biotechnology.
Significance of Structure
The significance of the double helix structure is profound; not only does it hold the key to genetic information, but it also allows for the precision of replication and transcription processes that are vital for life. The helical nature facilitates the packing of DNA within cells, allowing for efficient storage and retrieval of genetic information. The unique feature of this structure allows for interactions with various proteins, which are essential for processes such as gene expression and repair mechanisms.
Moreover, the double helix's resilience against damage and mutations ensures the long-term stability of genetic information across generations. This guarantees continuity in biological traits, underpinning the principles of heredity and evolution. Understanding this significance is paramount for those exploring the complexities of molecular biology.
Mechanisms of DNA Functioning
Understanding the mechanisms of DNA functioning sheds light on how genetic information is stored, replicated, and expressed in living organisms. Each of these processes plays a pivotal role in cellular activity, and knowing how they work helps to comprehend the very essence of life itself. The mechanisms involve intricate systems that ensure the reliability and precision of data being passed from one generation to the next.
Base Pairing and Complementarity
The way DNA strands interact through base pairing lays the foundation for several vital processes in biology. Remarkably, the two strands of the DNA double helix are held together through complementary base pairs, which play a crucial role in maintaining the structure of DNA while also facilitating its functions.
Adenine-Thymine Pairing
Adenine-thymine pairing stands out due to its unique characteristics. These two bases form two hydrogen bonds, which provide a moderate level of stability and complementarity. This simplicity allows for efficient pairing during DNA replication. The notable feature is that adenine, an aromatic amine, fits snugly with thymine, contributing to the overall organization of the DNA strand.
This pairing is a beneficial choice within the context of genomics because it contributes to the stability required for DNA to withstand environmental stresses. When looking through the lens of genetic diversity, its disadvantages may emerge as well. For instance, mutations can occur, impacting the reliability in certain scenarios, particularly when errors arise during replication.
Guanine-Cytosine Pairing
By contrast, guanine-cytosine pairing is characterized by its three hydrogen bonds, making it inherently stronger than adenine and thymine pairing. This robust structure is critical for maintaining the integrity of the DNA molecule under replication and transcription processes. The key advantage lies in its lesser tendency to undergo mutation since its stronger bonding helps secure genetic fidelity.
This pairing's unique feature is not merely mechanical; it reflects a biochemical elegance that enhances the resilience of certain regions within the DNA molecule. However, it can be seen as a disadvantage in a different light, as guanine-cytosine-rich areas can present challenges during replication due to their more complex structure.
DNA Replication Process
The DNA replication process is essential for cell division and ultimately affects everything from development to aging. This sophisticated mechanism ensures that genetic information is accurately copied and distributed among daughter cells. Each step is like a piece in a complex puzzle, working synergistically to achieve reliable replication.
Enzymatic Roles
In the DNA replication process, different enzymes perform specific functions critical to accuracy and efficiency. DNA polymerase is one of the primary enzymes, responsible for adding nucleotides to the growing DNA strand. It not only synthesizes new strands but also proofreads them, ensuring that any errors are corrected immediately. This function is crucial in maintaining genetic integrity, minimizing the occurrence of mutations.
Additionally, helicase unwinds the double helix, creating two single strands necessary for replication. Each enzymatic role enhances the overall fidelity of the replication process, allowing for an organized execution.
Replication Fork
The concept of the replication fork is an ingenious design that facilitates the replication process. At the fork, the DNA strands separate to form two single strands, allowing each to be replicated simultaneously. This architecture accelerates replication and simplifies the unwinding of the DNA.
The replication fork is beneficial for a couple of reasons: it allows for bidirectional replication, meaning that two new strands synthesize from each original strand at the same time. However, it can lead to problems if the replication machinery encounters obstacles like difficult sequences or damaged DNA.
In summary, the mechanisms of DNA functioning—through processes like base pairing, enzymatic roles, and replication architectures—underscore the elegance and intricate design that lies at the heart of biological information storage and transmission.
Role of DNA in Heredity
The role of DNA in heredity is like the blueprint for an elaborate structure, guiding what each part should be, how it should function, and how it relates to the whole. Understanding this part of DNA is crucial not only for geneticists or biologists but for anyone interested in the mechanisms of life itself. DNA carries genetic information from one generation to the next, making it the core of heredity and offering a glimpse into the characteristics passed down from parents to offspring.
Genetic Information Transmission
Genetic information transmission essentially involves the process through which traits are passed from parents to their children. This occurs through the intricacies of DNA replication during cell division, ensuring that the information needed to create proteins and regulate cellular functions is preserved and conveyed accurately.
A fundamental concept here is that each parent contributes half of the genetic material to their offspring. Thus, humans inherit 23 chromosomes from their mother and 23 from their father, amounting to 46 chromosomes that house thousands of genes. Each gene, in turn, determines specific traits, whether those are eye color, height, or even susceptibility to particular diseases.
Moreover, this transmission is not random; it follows certain patterns and rules, observable through family traits. For instance, when looking at traits like dimples or a widow’s peak, patterns emerge that often correlate closely with parental genotypes. This hereditary predictability lays the groundwork for many genetic studies and applications in medical science, underscoring how the DNA model serves as a linchpin in understanding health-related genetics.
Mendelian Genetics
Mendelian genetics, derived from the work of Gregor Mendel in the 19th century, builds on the foundation of heredity and adds layers of detail about how traits are inherited. Mendel’s experiments with pea plants illustrated that specific traits follow predictable patterns of inheritance. Through controlled cross-breeding of pea plants, he identified dominant and recessive traits, establishing what we now know as the laws of segregation and independent assortment.
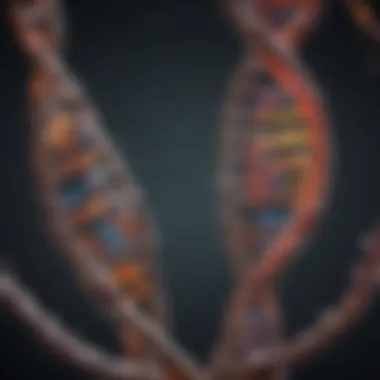
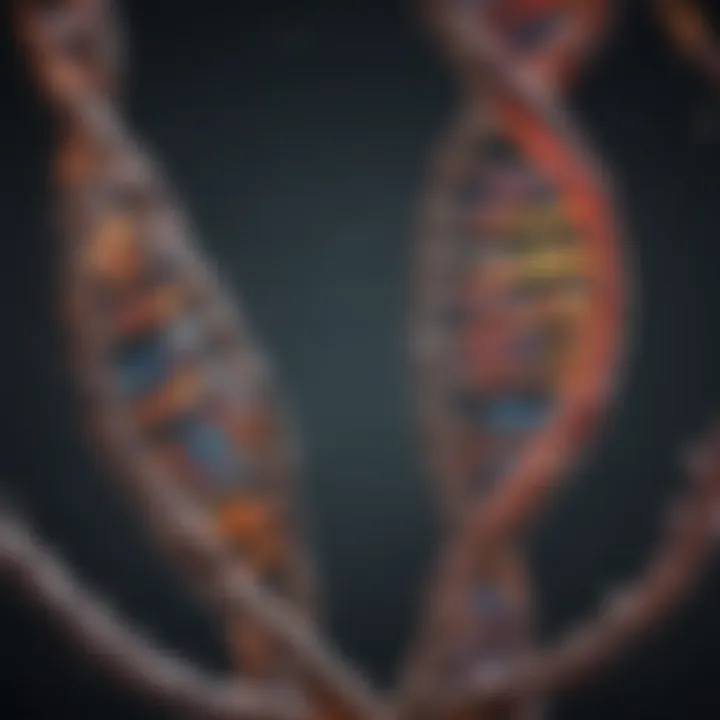
To break it down:
- Law of Segregation: During the formation of gametes, the pairs of alleles for a trait segregate, so that each gamete carries only one allele for each trait.
- Law of Independent Assortment: Genes for different traits can segregate independently during the formation of gametes.
These principles help explain complex traits and inheritance patterns observed in relatives. Mendelian inheritance lays the groundwork for understanding more complex forms of inheritance seen in polygenic traits and epigenetics, bridging a comprehension gap about how traits can manifest differently even within the same family.
"Genetic inheritance is a remarkable dance of probabilities, akin to tossing a pair of dice where both sequence and mix determine the outcome of each generation."
In summary, the role of DNA in heredity is about much more than just passing down traits; it's a comprehensive system that involves intricate processes and historical understanding of genetics. Without DNA, the very essence of heredity would not exist, highlighting the necessity of studying its structure and implications in shaping life.
Gene Expression and Regulation
Gene expression and regulation serve as cornerstones in the understanding of the DNA model. This intricate process governs how genes are turned on or off, thus shaping the functional landscape of an organism. Without gene expression, DNA would merely be a static repository of genetic information, lacking the dynamic capabilities essential for life. The ability to regulate which genes are expressed at a given time allows cells to adapt to changing environments, respond to signals, and maintain overall homeostasis.
Transcription and Translation
RNA Synthesis
RNA synthesis is a process crucial to gene expression. During transcription, an enzyme called RNA polymerase traverses the DNA strand, constructing a complementary RNA molecule based on the template provided by DNA. This synthesis is what ultimately converts the static information stored in DNA into a functional output. The key characteristic here is specificity; only certain genes are transcribed into RNA at any given time, determined by both internal and external signals.
A notable advantage of RNA synthesis is its efficiency in producing the molecules that facilitate cellular functions. However, it is worth considering that errors during transcription can lead to malfunctioning proteins or regulatory elements, which may have downstream effects on cellular machinery.
Protein Formation
Protein formation, synonymous with translation, takes the RNA produced during transcription and translates it into proteins using ribosomes. This conversion is physically where the magic happens, transforming genetic code into tangible action within the cell. The key trait of protein formation lies in its universality; virtually all cellular processes revolve around proteins.
One particular benefit of protein formation is that it allows for the diversity of functions within a cell. Different proteins can perform various roles, from enzymes that catalyze chemical reactions to structural components that give cells shape. However, if there is a misunderstanding of the RNA code or a misfolding in the proteins produced, it can lay the groundwork for diseases, underscoring the complexity of protein synthesis.
Regulatory Mechanisms
Promoters and Enhancers
Promoters and enhancers are critical elements in the regulatory landscape of genes. Promoters serve as landing sites for transcription machinery to initiate RNA synthesis, while enhancers amplify the activity of promoters, sometimes even from a distance. The unique feature of enhancers is their ability to regulate gene expression over considerable distances, allowing for a more nuanced control over when and how much gene is turned on.
The advantage of understanding these elements lies in their potential for biotechnological applications. Manipulating promoters and enhancers could lead to innovations in synthetic biology and genetic engineering. However, it's also worth noting that misregulation may result in diseases like cancer, emphasizing the necessity of precise control within these interactions.
Gene Silencing
Gene silencing refers to mechanisms that inhibit gene expression, ensuring that certain genes remain dormant when not needed. This is essential for cellular differentiation and development, allowing cells to specialize by silencing unnecessary genes. One key characteristic of gene silencing includes RNA interference, where small RNA molecules inhibit the translation of target mRNA.
In terms of strategy, gene silencing is a beneficial tool for researchers exploring gene function. By selectively silencing genes, scientists can uncover the roles of specific genes in various processes. Nonetheless, the potential for off-target effects poses a challenge, as unintended genes may also be silenced, leading to unpredictable outcomes in experiments or therapeutic approaches.
Gene expression and regulation not only underline the functional capacities of DNA but also open avenues for understanding complex biological systems and developing advanced methodologies in genetic research.
Modern Techniques in DNA Research
In the ever-evolving field of genetics, modern techniques in DNA research have been pivotal for advancements in science and medicine. These techniques enable researchers to manipulate and understand genetic material with a level of precision that was previously unimaginable. The implications of these methodologies reach across various domains, from healthcare to agriculture. In this section, we will explore two prominent techniques: Genetic Engineering and CRISPR-Cas9 technology, assessing their characteristics, applications, and ethical considerations.
Genetic Engineering
Genetic engineering refers to the direct manipulation of an organism's genes using biotechnology. This process allows scientists to alter DNA sequences and modify gene functions. One of the key aspects of genetic engineering is its ability to create genetically modified organisms (GMOs), which have become significant in both research and agricultural contexts.
Recombinant DNA Technology
Recombinant DNA technology is at the forefront of genetic engineering. This method involves combining DNA from different sources to create new genetic combinations. The beauty of this technology lies in its versatility: it can be used for diverse applications, from producing insulin for diabetes patients to developing crops resistant to pests. The key characteristic of recombinant DNA technology is its ability to create specific genetic changes that can enhance or modify traits of organisms.
The benefits of this technology are substantial, as it allows for targeted modifications rather than random changes found in traditional breeding techniques. However, it comes with its own set of disadvantages, such as possible unintended side effects on the organism or surrounding ecosystem.
Applications in Medicine
The applications of recombinant DNA technology in medicine have transformed the landscape of healthcare. This technique has made it possible to design targeted therapies and develop vaccines, which was particularly visible during the COVID-19 pandemic with the rapid development of mRNA vaccines.
One unique feature of applications in medicine is the ability to produce large quantities of therapeutic proteins. This characteristic has made it a notably beneficial choice for addressing various medical conditions at scale. However, it is essential to recognize that with these advancements also come ethical questions about safety, efficacy, and accessibility of such treatments to the public.
CRISPR-Cas9 Technology
CRISPR-Cas9 technology represents a breakthrough in DNA editing. It allows scientists to make precise alterations to the genome of organisms swiftly and efficiently. This technique has democratized gene editing due to its simplicity and affordability.
Mechanisms of Action
The mechanisms of action in CRISPR-Cas9 involve the use of a guide RNA that matches a specific DNA sequence in the genome. Once located, the Cas9 enzyme acts like molecular scissors, cutting the DNA at the desired location. This enables the incorporation of new genetic material or the disabling of undesirable genes. The primary characteristic making CRISPR-Cas9 a favored technique is its precision and ease of use.
Despite its promises, there are potential drawbacks, including the concerns about off-target effects where unintended parts of the genome might be altered, raising questions about long-term ramifications.
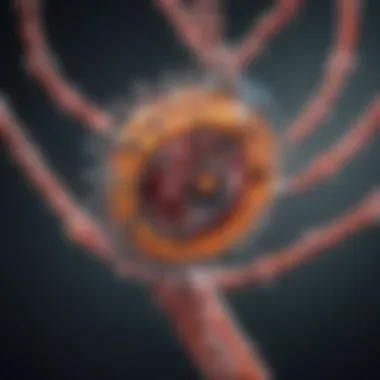
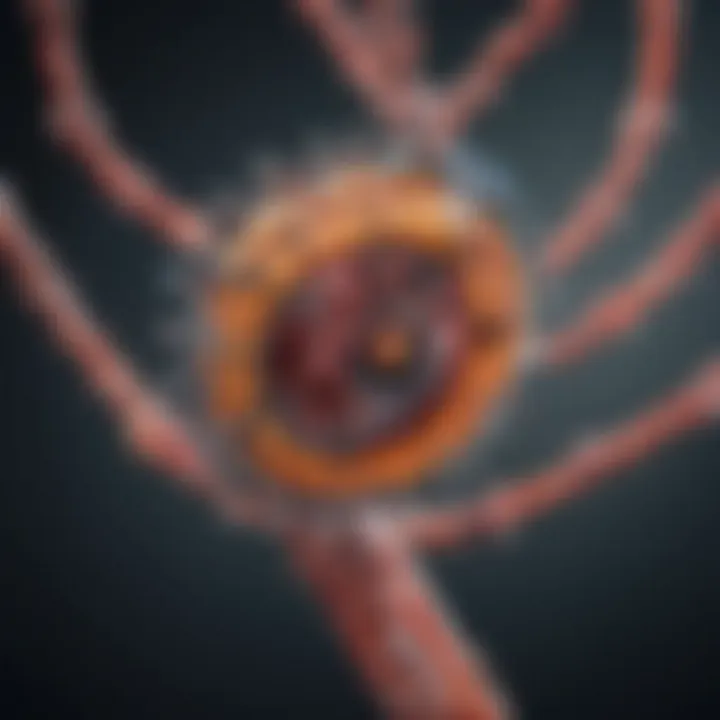
Ethical Implications
The ethical implications of using CRISPR technology are significant and complex. Discussions around its applications often revolve around concerns of gene editing in humans, particularly concerning inheritable traits. The key characteristic of these ethical implications is the requirement for dialogue and regulation. This ensures that advances in CRISPR technology are made responsibly.
One of the unique features of the ethical implications is the contrast between potential benefits, like eradicating genetic diseases, and moral dilemmas, such as designer babies. The advantages may be vast, but the disadvantages present compelling arguments against unrestricted applications of the technology, fostering heated debates in both scientific and public spheres.
"As we tread through the intricate world of gene editing, careful consideration of ethical boundaries is paramount."
In summary, modern techniques in DNA research like genetic engineering and CRISPR-Cas9 technology are reshaping our understanding and manipulation of genetic material. While they bring about revolutionary changes in medicine and agriculture, they also demand a thoughtful approach to ethical considerations, guiding us toward responsible use of these powerful tools.
Applications of DNA Science
DNA science has transformed numerous fields, shedding light on the inner workings of life itself. The applications of DNA research extend far beyond the laboratory, impacting medicine, agriculture, and various societal aspects. As we delve into this subject, we illuminate the advancements made possible through DNA knowledge, offering insights into the practical benefits and considerations that come with it.
Medical Implications
Gene Therapy
Gene therapy is a pioneering technique that aims to treat or prevent diseases by directly modifying genetic material within a patient’s cells. This approach has opened exciting doors in treating inherited disorders, some types of cancer, and viral infections. By targeting the root cause of illnesses at the genetic level, gene therapy stands out for its potential for a long-lasting impact on patient care.
The key characteristic of gene therapy is its ability to precisely target faulty genes, facilitating their repair or replacement. As a result, it presents a beneficial avenue for those suffering from chronic genetic conditions. For instance, conditions like cystic fibrosis or sickle cell disease have seen encouraging advancements with gene therapy, aiming to alleviate symptoms or even cure the affliction.
However, the technique is not without complications. While gene therapy offers a unique feature of potentially permanent solutions, concerns regarding ethical implications, unintended consequences, and long-term effects remain prominent. Balancing the advantages against the disadvantages is crucial as the field develops.
Personalized Medicine
Personalized medicine tailors medical treatment to individual characteristics, needs, and preferences. It takes into account factors like genetic makeup, environmental influences, and lifestyle to devise a strategy that best fits the patient. This emerging field relies heavily on DNA analysis to provide accurate information about how a patient may react to certain medications or therapies.
A standout feature of personalized medicine is its focus on understanding each person as unique. Such an approach results in treatments that correspond more effectively to individual health profiles, thereby enhancing patient outcomes. Patients are more likely to receive treatments that work for them and with fewer side effects, making it a popular choice in modern healthcare.
Nonetheless, there are challenges. The costs associated with the extensive testing required for personalized approaches can be prohibitive. Additionally, ethical considerations about data privacy and accessibility arise, requiring careful navigation. As the field progresses, balancing effectiveness and accessibility remains a key discussion point.
Agricultural Developments
Genetically Modified Organisms
Genetically modified organisms (GMOs) have taken the agricultural sector by storm, allowing scientists to alter the genetic makeup of crops for enhanced traits. From pest resistance to improved nutritional value, GMOs showcase how DNA science can tackle pressing agricultural challenges, such as food security and sustainability. The ability to enhance specific traits through genetic modification sets GMOs apart, making them a recognized choice for addressing critical agricultural needs. This technology can lead to higher yields and reduced reliance on chemical pesticides. However, it isn't all smooth sailing. There exists significant public concern regarding health risks and ecological impacts that GMOs might pose. Critics argue about potential allergenic effects and the unintended spread of modified traits to non-GMO crops, raising alarms that warrant careful examination as we adopt these scientific advances.
Sustainable Agriculture
Sustainable agriculture emphasizes methods that provide food without compromising future generations' ability to produce. The integration of DNA science is critical here as it enables the development of crops that require fewer resources while still delivering high productivity levels. This aspect of sustainable agriculture is particularly vital in the face of climate change, where conditions are becoming increasingly unpredictable. One unique feature of sustainable agriculture is its adaptability; it encourages using diverse planting strategies and crop rotations to promote soil health and reduce dependency on synthetic inputs. Thus, it becomes a practical choice within the modern agricultural landscape. Yet, challenges persist, including the need for comprehensive policies that support sustainable practices and educate farmers on genetic innovations.
Ethical Considerations in DNA Research
The field of DNA research has catapulted into the limelight, touching upon various facets of science, society, and ethics. As our understanding of genetic manipulation progresses, it becomes imperative to scrutinize the ethical considerations that accompany these revolutionary advancements. The implications of DNA research reverberate through medicine, agriculture, and even the very fabric of human identity. Thus, unpacking these ethical dilemmas is crucial for responsibly harnessing genetic technologies.
Concerns in Genetic Manipulation
Genetic manipulation, while offering robust solutions in healthcare and agriculture, raises numerous ethical concerns. These worries are not just the realm of science fiction; they manifest in real-world scenarios.
- Unforeseen Consequences: One major concern is the potential for unintended genetic mutations during manipulation. For instance, altering one gene might inadvertently affect others, leading to unforeseen health issues. The story of genetically modified crops recalls this concern, as some strains have ended up contributing to ecological imbalances.
- Long-term Effects: There’s also the question of long-term consequences. The field is still relatively new, and researchers are still determining how these alterations will play out over generations. Can the ecological web of life bear these changes? Will modified traits endure, or will they fade away when environmental conditions shift?
- Equity and Access: Another pressing issue is the concern for equity. Will access to cutting-edge genetic treatments be limited to affluent communities? The fear is that genetic advancements could widen the gap between those who have and those who do not. The thought of treatments being dictated by economic boundaries is troubling.
These are just a few threads that weave the intricate tapestry of ethical considerations surrounding genetic manipulation. Each thread adds depth and complexity to our understanding of what it means to 'play God' with genes.
Regulatory Frameworks
As the landscape of DNA research evolves, so too must the regulatory frameworks that govern it. Establishing robust guidelines is paramount in ensuring responsible scientific exploration.
- Legal Oversight: Striking a balance between innovation and safety is crucial. Many countries have initiated regulatory bodies specifically focusing on the ethical implications of genetic research. For example, the National Institutes of Health in the United States has strict guidelines on gene editing in human subjects, promoting a cautious approach that emphasizes informed consent.
- International Standards: Genetic research’s global nature necessitates collective agreements. Organizations like the World Health Organization are working towards establishing international norms that align various national regulations. Each country may have different perspectives on genetic modifications, complicating the regulatory landscape.
- Public Engagement: Involving the public in discussions about DNA research is also essential. This encompasses educating communities about potential benefits and risks, thus fostering informed opinions. Engaging in dialogue ensures that diverse voices contribute to shaping policies that govern genetic manipulation.
The conundrum of ethical considerations in DNA research isn’t simply black or white; it’s an intricate ballet of scientific promise and moral responsibility.
Through exploring these ethical concerns and regulatory frameworks, it becomes evident that while DNA research holds tremendous potential, it also demands a meticulous approach to navigate its challenges. Only through careful consideration can the scientific community harness the full power of DNA in ways that benefit society without compromising ethical standards.
Epilogue
The conclusion serves as the final note in our exploration of DNA, reinforcing the concepts discussed throughout the article while also pointing towards future avenues for research and inquiry. In this context, the significance can’t be overstated. Understanding the DNA model lays a solid foundation for advancements in various scientific fields including healthcare, genetics, and agriculture.
Future of DNA Research
Looking ahead, research into DNA offers a horizon filled with possibilities. Scientists are venturing into areas such as synthetic biology and personalized medicine, promising transformative approaches to treatment. The marriage of data science and gene editing tools like CRISPR is opening new doors. For instance:
- Gene Drives: Innovative uses of gene drives are intended for controlling pest populations and invasive species while maintaining ecological balance.
- Synthetic Genomics: The potential for creating entirely new organisms for uses ranging from biofuel production to pharmaceuticals is tantalizing.
Moreover, the integration of AI in genomics presents opportunities for faster discoveries. This combination may soon usher in a new age of predictive medicine, where the risk for disease can be assessed before symptoms even appear.
Summary of Key Insights
Throughout our discourse, several key insights have emerged:
- Molecular Architecture Matters: The structure of DNA isn't just a curiosity; it’s crucial for function. Understanding this allows scientists to apply this knowledge in biotechnology.
- Genetic Manipulation Tools: Modern techniques like CRISPR open up ethical conversations and practical possibilities that challenge how we think about genetic boundaries.
- Interdisciplinary Approaches: The blending of genetics with areas such as computer science and ethics is paving the way for holistic advancements in research and application.
The pursuit of knowledge surrounding DNA is akin to piecing together an intricate puzzle. Each fragment brings us closer to understanding the complexities of life. As we continue to unravel these mysteries, the implications for society, medicine, and ecosystems grow profoundly more significant.
"The more we discover about our DNA, the more we realize how interconnected everything truly is."
By grasping the nuances of DNA, we not only empower ourselves as scientists and learners but also promote a more informed conversation about the future of biotechnology and its ethical ramifications. Maintaining a vigilant approach to these discoveries is essential to ensure they’re used for the greater good.