Exploring Genetics and Heredity: Key Concepts Explained
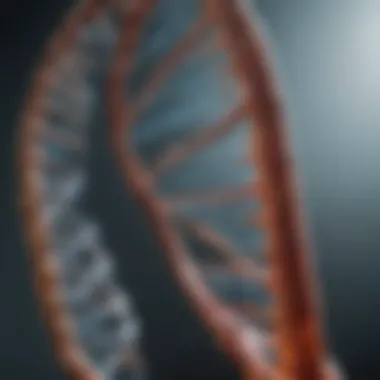
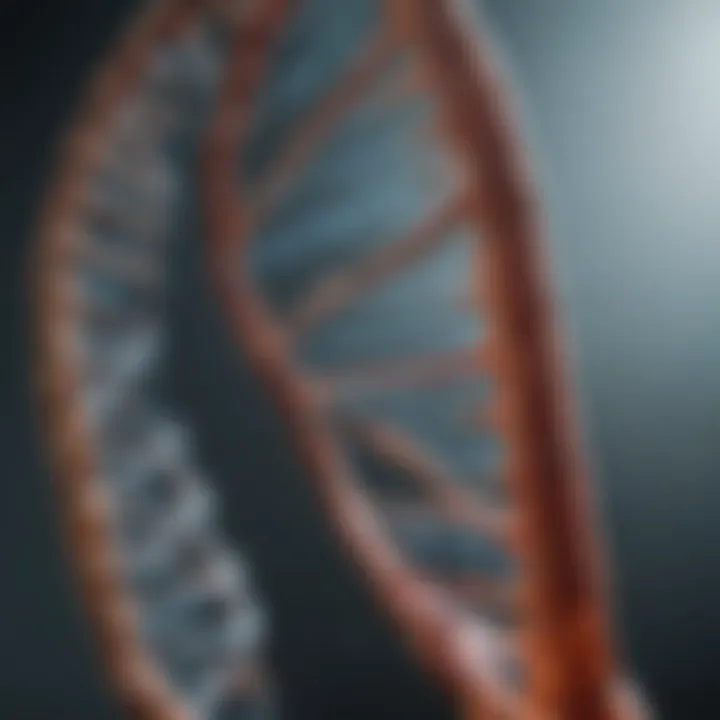
Intro
Genetics and heredity serve as the foundation of understanding biological diversity and organismal development. This realm of science investigates how traits are passed from parents to offspring, providing insights into everything from simple physical characteristics to complex disease susceptibilities. The study of genetics intertwines with various disciplines such as medicine, agriculture, and evolutionary biology, making it a crucial field of investigation in contemporary scientific discussions.
In an ever-evolving landscape, advancements in genetic research have sparked debates about ethical considerations related to gene editing, cloning, and genetic data privacy. By grasping the principles of genetics and heredity, one can better understand their implications on individual lives and societal structures. This exploration delves into these concepts, building a narrative that discusses the intricacies of DNA, gene expression, and trait inheritance.
The knowledge gained from this article caters to diverse audiences, including students, researchers, educators, and professionals, aiming to equip them with a profound comprehension of how genetics shapes not only personal identity but also population dynamics.
Research Overview
Summary of key findings
Genetic research reveals several crucial findings regarding DNA, gene function, and the inheritance patterns of traits. Here are some essential highlights:
- DNA Structure: Understanding the double helix model of DNA that was proposed by James Watson and Francis Crick greatly enhanced the study of genetics.
- Gene Expression: How genes are turned on or off has significant effects on development, health, and disease.
- Modes of Inheritance: Traits can exhibit complete dominance, incomplete dominance, or codominance, showcasing the complexity of genetic inheritance.
These discoveries lay a solid groundwork for further exploration into the realm of genetics.
Importance of the research in its respective field
The significance of genetics in modern science cannot be overstated. It informs various sectors:
- Medicine: Understanding genetic markers allows for advancements in personalized medicine. For example, certain cancers have identifiable genetic mutations that can guide treatment plans.
- Agriculture: Genetic tools, such as CRISPR technology, enhance the ability to develop crops that are resistant to pests and diseases.
- Evolutionary Biology: Genetic studies reveal how species evolve over time, showcasing the mechanisms of natural selection.
Together, these aspects establish genetics as a pivotal field that influences not only scientific inquiry but also practical applications affecting daily lives.
Preface to Genetics
The study of genetics is crucial for understanding how biological inheritance works. Genetics forms the foundation upon which much of modern biology rests. It is concerned with how traits are passed down from one generation to the next, and it provides insight into the mechanisms of heredity, which is essential for diverse fields such as medicine, agriculture, and evolutionary biology.
In this section, we will explore several key elements of genetics. First, we need to define what genetics is, establishing a baseline understanding of the discipline. Second, understanding genetics involves appreciating its historical context. This historical view allows us to realize how the field has evolved over time and how past discoveries continue to influence current research and applications.
Defining Genetics
Genetics is the branch of biology that deals with the heredity and variation of organisms. It involves the study of genes, genetic variations, and heredity in living organisms. The core unit of heredity is the gene, which is a segment of DNA that codes for specific traits. Genes dictate the biochemical processes in every cell, influencing aspects like eye color, height, and susceptibility to certain diseases.
Researchers in genetics analyze how traits are transmitted through generations, focusing on both dominant and recessive traits. The implications of these genetic foundations are vast, as they touch upon personal health, agriculture and even the evolution of species.
Historical Context
The history of genetics dates back to ancient times, but modern genetics began to take shape in the mid-19th century. Gregor Mendel, often referred to as the father of genetics, conducted experiments on pea plants that established the basic principles of heredity. His work highlighted how traits are inherited in specific patterns, which we now refer to as Mendelian inheritance.
Mendel's findings remained largely unrecognized until the early 20th century, when scientists like Thomas Hunt Morgan and others expanded upon these principles through experimental work with fruit flies.
As technology advanced, the discovery of DNA's structure in the mid-20th century transformed the understanding of genetics. James Watson and Francis Crick's iconic double helix model paved the way for molecular genetics, linking genetic information with cellular processes. This crucial point in history allowed researchers to understand gene expression, regulation, and the mechanics of genetic mutations.
Understanding DNA
Understanding DNA is fundamental to the study of genetics and heredity. DNA, or deoxyribonucleic acid, is the molecular blueprint that holds the instructions for the development, functioning, growth, and reproduction of all known living organisms. This section will explore the structure and replication of DNA, explaining their relevance in the broader context of genetics.
Structure of DNA
The structure of DNA is often described as a double helix. This unique configuration consists of two long strands of nucleotides wound around each other. Each nucleotide is composed of a phosphate group, a sugar molecule, and a nitrogenous base. There are four types of nitrogenous bases in DNA: adenine, thymine, cytosine, and guanine. The order of these bases encodes genetic information.
- Base Pairing: In the DNA double helix, adenine pairs with thymine, while cytosine pairs with guanine. This specific pairing is crucial for maintaining the integrity of the genetic information.
- Antiparallel Strands: The two strands of DNA run in opposite directions, which is described as being antiparallel. This orientation is important for the replication and transcription processes.
- Major and Minor Grooves: The twisting of the DNA strands creates major and minor grooves, which are essential for protein binding and the regulation of gene expression.
Understanding the structure of DNA allows for insights into how genetic information is stored and retrieved, making it a cornerstone in genetics research.
Replication Process
DNA replication is the process by which a cell duplicates its DNA before cell division. This ensures that each daughter cell receives an exact copy of the DNA. The replication process can be broken down into several key steps:
- Initiation: The DNA double helix unwinds at specific locations called origins of replication.
- Unwinding: Enzymes called helicases separate the two strands of DNA, creating a replication fork. This exposes the nitrogenous bases for pairing.
- Complementary Base Pairing: Free nucleotides in the nucleus pair with the exposed bases on each strand. DNA polymerase is the enzyme responsible for adding nucleotides to the growing strand.
- Formation of New Strands: As nucleotides are added, complementary strands are formed. This produces two identical DNA molecules, each composed of one original strand and one newly synthesized strand, a process known as semiconservative replication.
- Termination: The replication process continues until the entire molecule is copied. The resulting DNA strands then undergo proofreading to correct any errors that may have occurred during replication.
"DNA replication is the fundamental process by which genetic information is transmitted from cell to cell and from generation to generation."
The understanding of DNA replication is crucial as it lays the foundation for various genetic applications, such as gene therapy and cloning, and it informs the study of genetic diseases related to replication errors.
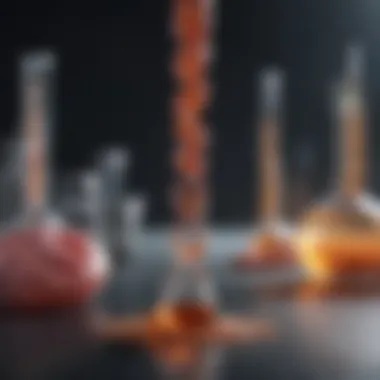
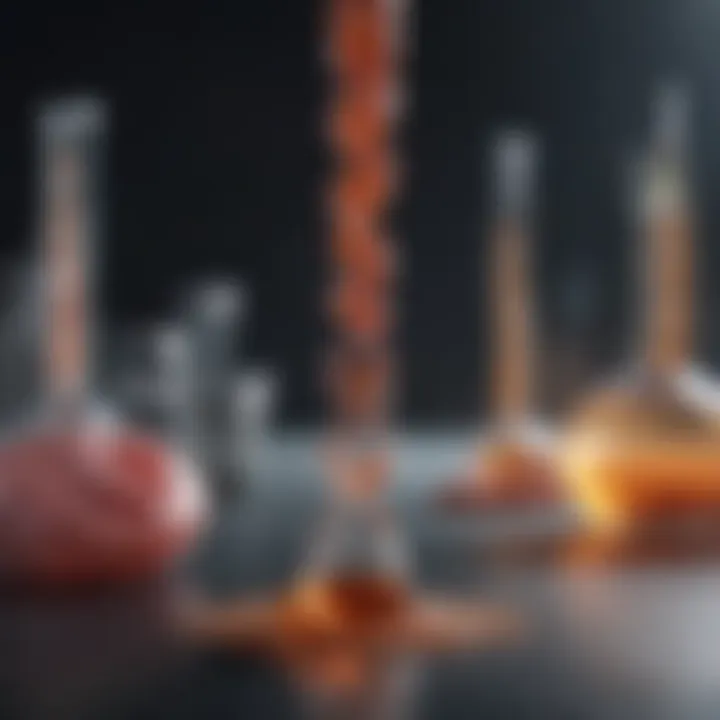
Gene Expression
Gene expression is a fundamental biological process that governs how genetic information is converted into functional products, primarily proteins. Understanding gene expression is essential because it links the genetic information inherited from an organism's ancestors to the physical, observable traits that emerge. This section will cover the critical processes of transcription and translation and the regulatory mechanisms that control these processes.
Transcription and Translation
Transcription and translation are the two key stages in gene expression. During transcription, the information in a gene's DNA is copied to produce messenger RNA (mRNA). This occurs in the nucleus of the cell and involves several key players, including RNA polymerase. RNA polymerase unwinds the DNA and synthesizes a strand of mRNA complementary to the DNA template. This mRNA strand then undergoes processing, such as capping and polyadenylation, before leaving the nucleus.
Once the mRNA reaches the cytoplasm, the process of translation begins. Here, ribosomes read the mRNA sequence in sets of three nucleotides, known as codons. Transfer RNA (tRNA) brings the corresponding amino acids to the ribosome, where they are joined together, forming a polypeptide chain. This chain subsequently folds into a functional protein that may serve structural, enzymatic, or regulatory roles in the cell.
"Gene expression is not just a simple linear process; it is a complex web of interactions and regulations that ultimately shapes the phenotype of an organism."
Regulatory Mechanisms
Regulatory mechanisms play a crucial role in gene expression, determining when and how genes are expressed. These mechanisms ensure that proteins are produced at the right time and in the right amounts, allowing cells to respond effectively to internal and external signals. There are several layers of regulation in gene expression:
- Transcription Factors: Proteins that bind to specific DNA sequences to enhance or inhibit transcription. They can be classified into general transcription factors, which are essential for all gene transcription, and specific transcription factors, which regulate particular genes.
- Epigenetic Modifications: Chemical changes to DNA or histone proteins can influence gene expression without altering the underlying DNA sequence. For example, methylation of DNA can silence genes, preventing their transcription.
- RNA Processing: Before mRNA is translated into protein, it may be spliced to remove introns and join exons, sometimes leading to the production of different protein isoforms from a single gene.
- Control of Translation: Regulatory proteins can bind to mRNA, influencing its stability and translation efficiency. This kind of control is vital for rapid responses to changing cellular environments.
In summary, gene expression is a multi-step process that not only involves the fundamental processes of transcription and translation but also depends significantly on various regulatory mechanisms. Understanding these elements is vital for appreciating how genes govern cellular function and influence traits across generations.
Mendelian Genetics
Mendelian genetics is a crucial framework within the broader study of genetics. Named after Gregor Mendel, who pioneered the science of heredity in the mid-19th century, this field provides the foundations for understanding how traits are inherited through generations. The principles that Mendel uncovered laid the groundwork for modern genetics, making it an essential topic in this article.
Laws of Inheritance
Mendel articulated the Laws of Inheritance, which consist of three primary principles:
- Law of Segregation: This law states that allele pairs separate during the formation of gametes, meaning each gamete receives only one allele from each pair.
- Law of Independent Assortment: Mendel observed that alleles for different traits are distributed to sex cells (<gametes>) independently of one another. This independence leads to genetic variation in offspring.
- Law of Dominance: According to this law, some alleles are dominant, and others are recessive. If an organism has at least one dominant allele, that trait will be expressed in its phenotype.
These laws underscore the predictable patterns of inheritance and aid in understanding how traits can manifest in offspring. Their relevance transcends biology, influencing fields like medicine, agriculture, and conservation. For instance, utilizing these principles, scientists can predict genetic outcomes in breeding programs.
Punnett Squares
Punnett squares are a practical tool in Mendelian genetics, allowing researchers and students to visualize genetic crosses. They illustrate how alleles combine and predict the probability of an offspring displaying a particular phenotype.
A simple Punnett square can be constructed as follows:
- Take two parent organisms and identify their genotypes. For example, consider one parent with genotype Aa (heterozygous) and another with genotype aa (homozygous recessive).
- The Punnett square is drawn with one parent's alleles along the top and the other's along the side:
From this simple example, the probabilities of the offspring's genotypes can be derived. There is a 50% chance for Aa and a 50% chance for aa. Such analysis is valuable in genetic counseling and breeding strategies, providing insight into inheritance patterns and likelihoods.
"Understanding Mendelian genetics is essential for grasping the principles that govern inheritance, impacting both theoretical and applied scientific contexts."
In summary, the study of Mendelian genetics serves as a key foundation in genetics, aiding various scientific disciplines and offering tools like Punnett squares for genetic analysis. By understanding inheritance patterns, researchers can make informed predictions and decisions relevant to evolution, population genetics, and beyond.
Types of Inheritance
Understanding the various types of inheritance is crucial for comprehending how traits are passed from parents to offspring. It provides insight into genetic disorders, evolutionary biology, and the applications of genetic engineering in modern science. By examining different inheritance patterns, we can better predict phenotypic outcomes in offspring and understand the complexities of genetic expression and variation.
Autosomal Dominant and Recessive Traits
Autosomal dominance and recessiveness refer to patterns of inheritance linked to alleles of genes located on non-sex chromosomes. In autosomal dominant inheritance, only one copy of a mutated gene is sufficient to express the trait. For například, if one parent has Huntington’s disease, there is a 50% chance their child will also inherit the disorder. On the contrary, autosomal recessive inheritance requires two copies of the mutated gene for the trait to manifest. Taysachs disease is an example. If both parents are carriers, their child has a 25% chance of expressing the disease.
The benefits of understanding these types include the ability to assess risk factors for certain conditions and the impact on family planning. Genetic testing can reveal whether an individual carries a recessive allele, which can be particularly important for couples planning to have children.
X-linked Inheritance
X-linked inheritance pertains to genes located on the X chromosome. Because males have only one X chromosome, they are more likely to express X-linked traits. For instance, color blindness predominantly affects males, since they inherit only one X chromosome from their mother. In contrast, females have two X chromosomes and can be carriers without showing symptoms, reducing their risk of expressing X-linked disorders.
This type of inheritance is vital for understanding specific genetic conditions, such as hemophilia and Duchenne muscular dystrophy. Prenatal testing and genetic counseling are essential tools for assessing the likelihood of passing down these traits, especially in families with known X-linked disorders. Furthermore, healthcare professionals must consider the implications of X-linked traits in both diagnosis and treatment.
Mitochondrial Inheritance
Mitochondrial inheritance, also called maternal inheritance, refers to the transmission of genes located in mitochondria, the energy-producing organelles within cells. Since mitochondria are inherited from the mother, all offspring will potentially inherit mitochondrial traits. Disorders such as Leber’s hereditary optic neuropathy are passed down exclusively through maternal lines.
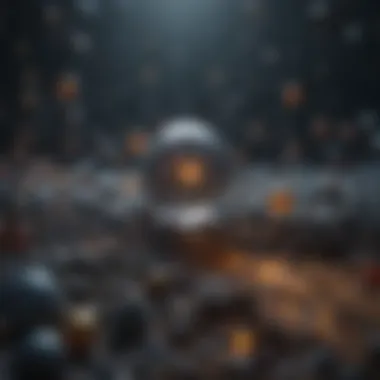
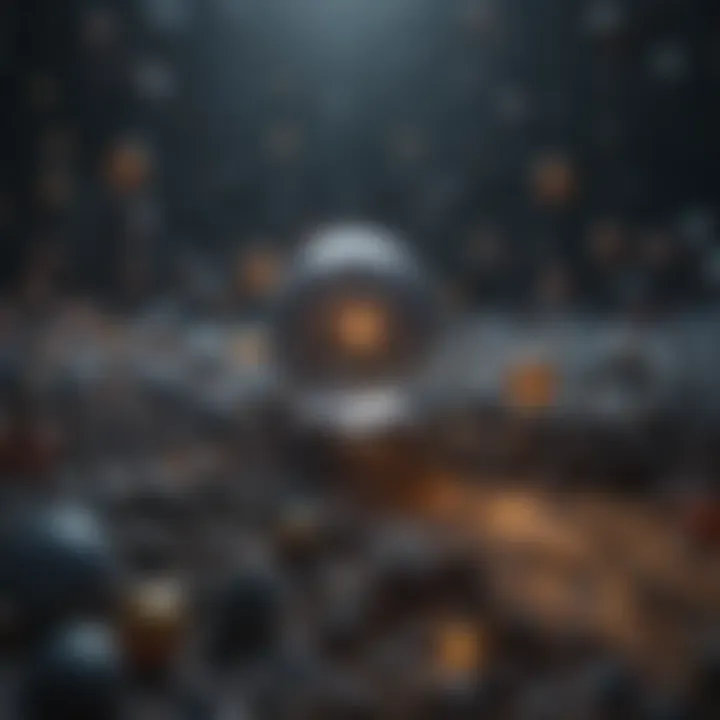
Understanding mitochondrial inheritance is important not only for tracking certain diseases but also for exploring the origins of certain traits. It raises significant questions about the roles of mitochondrial DNA in complex disorders and the potential for therapies targeting mitochondrial dysfunction. Mitochondrial analysis can thus provide insights into energy metabolism diseases and is a growing area of research in genetics.
In summary, different types of inheritance shape our understanding of genetics. They reveal patterns that are essential for genetics and heredity studies, especially when considering family health histories and potential genetic disorders. Understanding these patterns can have profound implications for medical research and public health.
Genetic Variation
Genetic variation refers to the diversity in gene frequencies among individuals within a population. This variation is crucial as it affects how a species can adapt to environmental changes and survive over time. The understanding of genetic variation enables research in various fields, particularly in medicine, agriculture, and conservation. In the context of genetic research, recognizing the sources of genetic variation and its implications plays a key role in various applications.
Sources of Genetic Variation
There are several mechanisms that contribute to genetic variation within a population. These include:
- Mutation: Mutations are permanent changes in the DNA sequence. They can occur spontaneously or be induced by environmental factors. These changes create new alleles, introducing new genetic material into a population.
- Gene Flow: This refers to the transfer of genetic material between populations. When individuals migrate and interbreed, they contribute their genetic material to the new population, enhancing genetic diversity.
- Genetic Recombination: During sexual reproduction, genes from two parents combine. This process generates offspring with a unique combination of genes. This is particularly evident during meiosis, where chromosomes exchange segments before being passed on to gametes.
"Genetic variation is the foundation for evolution and the survival of species, acting as a reservoir of potential adaptations."
These sources of genetic variation are vital for evolutionary processes and help maintain the stability of populations over time.
Population Genetics
Population genetics is the study of genetic variation within populations, and it examines how gene frequencies change over generations. Understanding population genetics is essential for comprehending evolutionary mechanisms.
Here are some core principles:
- Allele Frequency: The proportion of a particular allele among all allele copies in a population. Tracking changes in allele frequency helps gauge evolutionary changes.
- Hardy-Weinberg Principle: This principle provides a baseline for studying genetic variation. It suggests that allele and genotype frequencies will remain constant in a population under specific conditions (no mutation, random mating, no natural selection, etc.).
- Genetic Drift: Random fluctuations in allele frequencies, more pronounced in smaller populations. It can lead to the loss of genetic variation.
Population genetics helps researchers understand how evolution shapes the genetic structure of populations, providing insights into how species adapt to their environments.
In summary, genetic variation is crucial for understanding inheritance and evolution. By exploring its sources and studying population genetics, researchers can gain insights into the mechanisms underlying biodiversity.
Advances in Genetic Engineering
Advances in genetic engineering have revolutionized the way we understand and manipulate biological systems. This field combines the principles of genetics, molecular biology, and biotechnology to alter organisms' genetic makeup. The significance of these advancements cannot be overstated, as they provide powerful tools for a range of applications in various domains, including medicine, agriculture, and more. The ability to edit genomes accurately has given rise to numerous possibilities in both scientific research and practical applications, addressing some critical challenges facing society today.
One key element is the precision associated with genetic modifications. Traditional methods often resulted in random mutations, making it difficult to predict outcomes or ensure uniformity. The development of advanced techniques, particularly CRISPR technology, allows for targeted changes to specific genes, leading to more reliable and effective results. This precision not only enhances the potential for research but also plays a crucial role in developing treatments for genetic disorders.
In addition to its precision, genetic engineering holds several benefits:
- Improved crop yields and resistance to diseases in agriculture.
- Development of specialized medical therapies tailored to individual patients.
- Potential to create engineered organisms for environmental cleanup tasks.
However, ethical considerations abound in this field. The manipulation of genes raises questions concerning the implications of altering hereditary information and the long-term effects on ecosystems and human health. This space is one that requires ongoing careful consideration and discussion among scientists, ethicists, and the broader public.
"With great power comes great responsibility." This adage is particularly applicable in the context of genetic engineering, where the potential consequences of scientific decisions can be profound.
CRISPR Technology
CRISPR technology represents a breakthrough in genetic engineering. It stands for Clustered Regularly Interspaced Short Palindromic Repeats. Initially discovered in bacteria, it functions as a defense mechanism against viruses. This discovery has led to the adaptation of CRISPR as a tool for editing genomes in various organisms.
The mechanics of CRISPR involve making deliberate cuts in the DNA to add, remove, or alter genetic material. The efficiency and simplicity of this method make it highly accessible and cost-effective compared to previous techniques. Researchers can now conduct experiments with unprecedented speed and accuracy, which promotes innovation across different fields.
The implications of CRISPR technology extend well beyond basic research. They range from agriculture, where crops can be engineered to possess desirable traits, to medicine, where it can be used to target and correct genetic disorders before they manifest.
Applications in Medicine
The applications of genetic engineering, particularly through CRISPR, are reshaping the medical landscape. Genetic therapies are being developed to address a variety of conditions previously thought untreatable. For instance, sickle cell anemia is one significant focus where gene editing is being explored to correct the genetic mutations that cause this painful disorder.
Additionally, cancer treatment is evolving with the integration of genetic engineering. By modifying immune cells to better target and destroy cancer cells, researchers are developing innovative therapies that customize treatment based on individual genetic profiles. This personalization enhances the effectiveness of treatments, potentially improving patient outcomes.
A few notable applications include:
- Gene therapy: Tools like CRISPR are employed to replace or deactivate faulty genes.
- Vaccination: Genetic modifications could lead to more effective vaccines, particularly against viral infections.
- Regenerative medicine: Stem cell research is benefitting from genetic modifications, allowing for more precise therapies and restoration of damaged tissues.
Ethical Considerations in Genetics
The field of genetics has transformed significantly in recent years, making it imperative to deliberate on various ethical considerations. The implications of genetic research extend beyond scientific discoveries. They affect both individual rights and societal norms. As we unlock the secrets of DNA, the responsibility to navigate the associated ethical landscape grows.
Firstly, ethical considerations help ensure that advancements do not lead to misuse of genetic data or exploitation of individuals. Genetic information can reveal sensitive health data. This raises concerns about privacy and consent. Protecting genetic privacy means secure storage and sharing protocols.
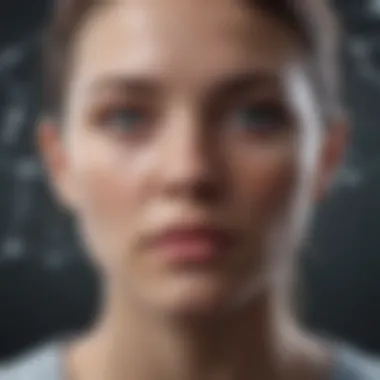
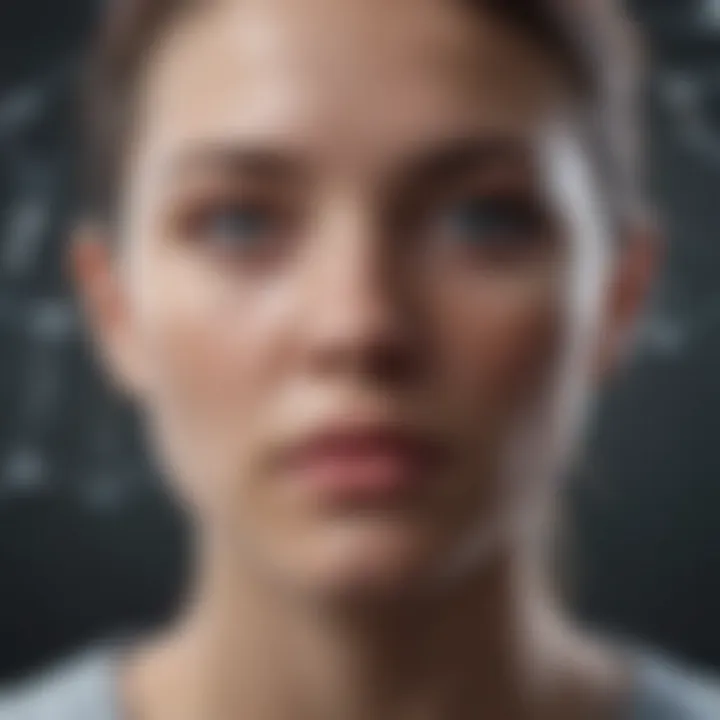
Furthermore, understanding ethical concerns can help prevent discrimination based on genetic traits. People may be treated differently based on inherited characteristics. Keeping genetic testing accessible without stigma is crucial. This promotes equal opportunities for all. Effective policies must be developed to avoid discrimination in employment or insurance based on genetic predispositions.
Genetic Privacy
Genetic privacy stands as a cornerstone of ethical considerations. The collection of DNA data opens doors to insights that were previously unreachable. However, it also introduces risks for privacy breaches. If individuals' genetic data is not managed correctly, it could be used in harmful ways. An important point to ponder is the balance between research benefits and individual rights.
Privacy laws surrounding genetic information must be robust. They should establish clear guidelines on who can access this data. Moreover, individuals must have control over their genetic information. Informed consent is essential, ensuring that people understand how their data will be used.
- Individuals should:
- Know what genetic information is collected.
- Understand the potential consequences of sharing their data.
- Choose whom to share their data with willingly.
Security measures must evolve alongside technology. Protecting genetic data from unauthorized access is paramount to building trust in genetic research. Researchers and institutions must prioritize confidentiality. This ensures individuals can participate in studies without fear.
"The right to control personal genetic information is fundamental to maintaining individual autonomy."
Designer Babies and Eugenics
Designer babies refer to the concept of using genetic engineering to select specific traits in offspring. This raises significant ethical questions. The potential for eugenics, selected breeding to enhance particular traits, harkens back to troubling historical precedents. A core issue is the morality of altering human genetics fundamentally.
The prospect of designer babies can lead to societal divides. Those with access to genetic enhancements may have advantages. This could create a new form of inequality. The concept should be approached with caution, balancing potential benefits with ethical responsibilities.
Moreover, unintended consequences merit attention. Editing genes might lead to unforeseen effects not observed in current studies. Assessing long-term impacts is vital. Most importantly, prioritizing health and welfare over superficial enhancements should govern these decisions.
- Considerations regarding designer babies include:
- The implications of genetic modifications on future generations.
- Potential societal divides based on access to genetic enhancement.
- Ensuring ethical frameworks guide research and application of genetic technologies.
Role of Genetics in Evolution
The role of genetics in evolution is crucial for understanding how species adapt and change over time. This section will discuss how genetic variation contributes to evolutionary processes, the mechanisms of natural selection, and how genetic drift can impact populations. Each aspect relates directly to the larger framework of heredity and species development, making their study essential for students and professionals in biology and related fields.
Natural Selection
Natural selection is one of the most well-known mechanisms of evolution. It describes the process through which traits become more or less common in a population based on their impact on survival and reproduction. For example, in a given environment, individuals with favorable traits are more likely to survive and reproduce, passing those traits to the next generation. Over time, this leads to changes in the genetic makeup of a population.
Key factors that contribute to natural selection include:
- Variation: Individuals in a population exhibit different traits, which can be inherited.
- Competition: Resources such as food, mates, and habitat are limited, causing individuals to compete.
- Survival of the Fittest: Those best adapted to their environment are more likely to thrive and reproduce.
Natural selection can drive significant changes, such as the development of antibiotic resistance in bacteria. This is a stark reminder of how quickly evolution can occur, especially under intense selective pressure.
Genetic Drift
Genetic drift is another essential evolutionary mechanism, distinct from natural selection. It refers to random changes in allele frequencies within a population due to chance events. This can lead to significant evolutionary changes, especially in small populations. For instance, if a natural disaster randomly eliminates a large portion of a population, the genetic traits of the survivors will dictate the genetic composition of future generations.
Key points about genetic drift include:
- Random Events: Unlike natural selection, genetic drift is influenced by random factors, not by the suitability of traits.
- Bottleneck Effect: When a population's size is drastically reduced, genetic diversity decreases, potentially leading to extinction.
- Founder Effect: If a small group establishes a new population, their genetic traits can dominate the new group's genetic structure.
Both natural selection and genetic drift work concurrently, shaping the evolutionary paths of various species. To understand
Epilogue
In summary, the conclusion section encapsulates the vital insights derived from an extensive discussion of genetics and heredity. It serves as a reflective surface, distilling the significant principles and findings highlighted throughout the article. Understanding genetics is not only foundational for the scientific community but also crucial for broader societal implications. The intricate mechanisms of inheritance shape both individual traits and population dynamics, influencing everything from health to personal identities.
This article emphasizes the importance of recognizing the dual nature of genetic advancements. While they present tremendous opportunities for improving healthcare and agriculture, they also prompt ethical discussions. Addressing these aspects is essential in pursuing future genetic research, ensuring that developments serve the common good without compromising moral standards.
"The study of genetics is at the forefront of scientific discovery, influencing various disciplines and practical applications."
As we move toward an era where genetic knowledge continues to expand, the relevance of understanding this field remains paramount. The potential for breakthroughs in treatments for diseases, coupled with the responsibility to navigate ethical lines, poses a unique challenge for scientists, researchers, and policymakers alike.
Summary of Key Points
In this exploration of genetics and heredity, several essential points emerge:
- Foundational concepts: A clear understanding of DNA structure, gene expression, and inheritance laws.
- Human impact: The implications of genetic research in medicine and beyond.
- Ethical considerations: The emerging discussions around privacy, designer genetics, and societal norms.
- Evolutionary perspective: How genetic variation and natural selection influence species.
These elements collectively provide a framework for understanding the complexities of genetics, encouraging critical thinking about current and future implications in various fields.
Future Directions in Genetic Research
Looking ahead, the future directions in genetic research are vast and filled with potential. Several key areas promise to reshape our understanding and application of genetics:
- Gene Therapy: Continued advancements in gene editing technologies, such as CRISPR-Cas9, are likely to transform approaches to treating genetic disorders.
- Personalized Medicine: Research will enhance capabilities for tailoring medical treatments based on individual genetic profiles, improving patient outcomes.
- Synthetic Biology: The creation of new biological parts and systems could lead to groundbreaking applications in agriculture, energy, and medicine.
- Genomic Data Privacy: As genetic testing becomes more commonplace, the need for strong frameworks that protect individual genetic information will grow.
- Population Genomics: Understanding the genetic variations within populations will be essential for addressing public health and evolutionary questions.