In-Depth Study of Electronic Flow in Science

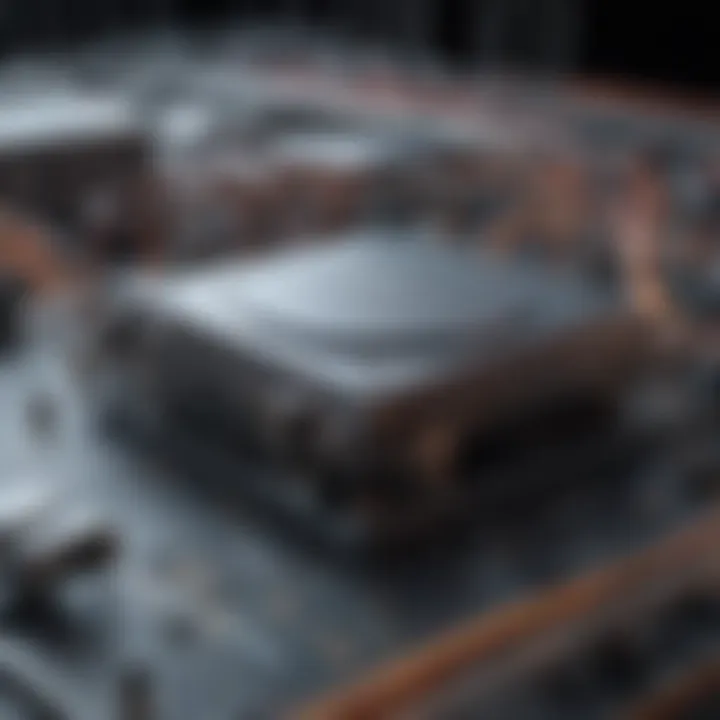
Intro
In recent years, the concept of electronic flow has gained increasing attention across multiple scientific fields. From physics to biology, this multifaceted subject has shown itself to be the backbone of many experiments and applications. Understanding electronic flow not only enhances our comprehension of various phenomena but also opens doors to new technologies and innovations.
Electronic flow revolves around the movement of electrons through different mediums. This flow plays a crucial role in several processes, including energy transfer, chemical reactions, and biological functions. Grasping the fundamental principles of electronic flow is key for students and researchers alike, as they navigate the intertwining threads of theory and application in their respective disciplines.
As we embark on this comprehensive analysis, it’s essential to lay a solid foundation by examining the core elements of electronic flow. This will create a robust framework from which we can explore its importance, mechanisms, applications, and the challenges it presents in contemporary scientific inquiry.
Research Overview
Summary of Key Findings
This exploration into electronic flow reveals several vital insights:
- Foundational Theories: Investigating the fundamental principles of electronic flow helps clarify its behavior in various contexts. Quantum mechanics, for instance, offers significant theories that enlighten this phenomenon, showcasing how electrons interact with their environments.
- Diverse Applications: Electronic flow is ubiquitous; it underpins technologies from batteries and transistors to biological systems and photosynthesis. Its scope ranges widely across disciplines.
- Contemporary Challenges: The study of electronic flow is not without hurdles. Issues such as energy efficiency, miniaturization in circuits, and environmental impacts of electronic waste remain pressing concerns that require innovative approaches.
Importance of the Research in its Respective Field
Grasping the dynamics of electronic flow is fundamentally important. Not only does it provide clarity in fields like physics and chemistry, but it also allows for informed developments in technology and environmental science. Educational resources that unravel this complexity can motivate future scientists, helping them appreciate the relevance of these concepts in shaping our world.
"Understanding electronic flow is akin to unlocking the secrets of nature's playground!"
- Renowned Physicist
Methodology
Description of Experimental or Analytical Methods Used
To analyze electronic flow, various methodologies have been employed:
- Electrochemical Measurements: Techniques like cyclic voltammetry offer insights into electron transfer processes, allowing researchers to quantify the flow.
- Spectroscopic Techniques: Methods such as spectroscopy help visualize the interactions of electrons with different materials, illustrating how flow is altered by varying conditions.
Sampling Criteria and Data Collection Techniques
Appropriate sampling is essential in studying electronic flow. Researchers often focus on:
- Selecting materials that exhibit distinct electronic properties.
- Ensuring a broad representation of data collected across different environments and conditions, allowing for a comprehensive understanding of the flow dynamics.
Through rigorous methodologies, the rich landscape of electronic flow can be better illuminated, paving the way for future exploration and innovation.
Defining Electronic Flow
Understanding electronic flow is crucial to grasp various scientific concepts that rely on the movement of electrons, ions, and charge carriers. While often seen as a mere technicality within physics, its relevance spans across chemistry, biology, and even engineering. From the way a light bulb illuminates to the complex reactions in living cells, electronic flow forms the backbone of many phenomena we observe in our daily lives. It encompasses both the fundamental principles that govern charge movement and the real-world applications of these principles.
As we delve deeper into electronic flow, it’s pertinent to consider some key elements:
- Charge Movement: This is the essence of electronic flow. It's about how charges travel through a medium, which can significantly affect the conductivity of materials.
- Energy Transfer: Electronic flow is not just about movement; it's also about energy transfer that occurs alongside this movement, impacting everything from electronic devices to biochemical processes.
- Applications in Technology: In today's digital age, understanding electronic flow is crucial for advancements in technology, from semiconductors to renewable energy solutions.
The importance of defining electronic flow lies in its multifaceted nature, as this understanding allows us to innovate, create, and solve challenges across various domains. Now, let's travel back in time to see how the concept has evolved.
Historical Context
The journey of electronic flow can be traced back to key experiments and ideas that helped pave the way for modern science. In the early 19th century, scientists like Alessandro Volta and Michael Faraday laid the groundwork for understanding electricity. Volta's invention of the voltaic pile was pivotal in demonstrating how chemical reactions could produce electrical energy. Faraday's contributions, particularly in electromagnetism, highlighted the relationship between electricity and magnetism, furthering the understanding of electronic flow.
Moreover, discoveries in the 20th century, such as quantum mechanics, reshaped our comprehension of charge carriers at a microscopic level. The development of semiconductor technology in the mid-20th century has revolutionized electronic flow from labs into everyday devices. This rich historical context is vital for appreciating how we got to where we are today and why understanding electronic flow continues to be significant.
Fundamental Principles
At the core of electronic flow are several fundamental principles that describe how electronics behave. These principles not only explain the movement of electrons but also their interactions within different materials. Here are a few key aspects:
- Ohm's Law: This principle states that the current flowing through a conductor between two points is directly proportional to the voltage across the two points and inversely proportional to the resistance between them. It's a cornerstone of circuit theory and a great way to understand basic electronic flow.
- Charge Carriers: In metals, electrons are the primary charge carriers, while in electrolytes, it can be ions. Understanding the type of charge carrier is essential, because it affects how materials conduct electricity.
- Conductivity Types: Conductors, insulators, and semiconductors each behave differently when it comes to electronic flow. For instance, semiconductors can conduct electricity under certain conditions, making them crucial in countless modern applications, especially in electronics.
These principles not only define electronic flow but also frame the discussion for its broader implications in various scientific fields and technologies.
"Understanding the flow of electrons is more than academic; it plays a vital role in everyday technology and biological processes."
By examining these foundational ideas, readers can appreciate the intricate balance and behaviors that govern electronic flow, which in turn enhances our approach to both research and application in the field.
The Role of Electronic Flow in Physics
Electronic flow is at the heart of many physical phenomena. Understanding how electrons navigate through materials informs a wide range of scientific and practical applications. It plays a pivotal role in the realms of electricity, magnetism, and even thermodynamics. This section will dissect the fundamental elements of charge and current flow, as well as the implications of circuit theory in the physics arena.
Charge and Current Flow
At its core, electronic flow encompasses the interplay of charged particles. When a potential difference – or voltage – is applied across a conductor, electrons, which carry a negative charge, begin to drift towards areas of higher potential. This movement creates what we call current. The relationship between voltage (V), current (I), and resistance (R) is encapsulated by Ohm's Law, which states:
I = V/R
This formula is the bedrock of understanding how electrical circuits operate. However, delving deeper into charge flow brings to light more nuanced phenomena such as drift and diffusion currents. Drift current is the intentional, directional flow of charge due to an electric field, whereas diffusion current refers to the movement of charge carriers caused by concentration gradients.
In practical terms, different materials display varying degrees of conductivity. Metals like copper exhibit high conductivity, allowing electrons to flow with minimal resistance, while insulators like rubber restrict the movement of charge. In understanding these variations, we can design more efficient electronic systems and optimize energy transfer.
Circuit Theory Implications
Circuit theory leverages the principles of electronic flow to model and analyze complex electrical networks. The driving question at the heart of circuit theory is often: "How can components work together to perform a desired function?" By applying Kirchhoff's laws — Kirchhoff’s current law (KCL) and Kirchhoff’s voltage law (KVL) — engineers can predict how currents and voltages distribute throughout a circuit.
Kirchhoff’s current law states that the total current entering a junction equals the total current leaving the junction.
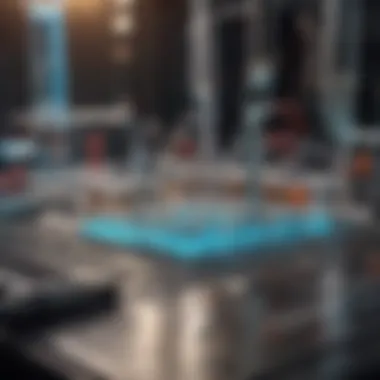
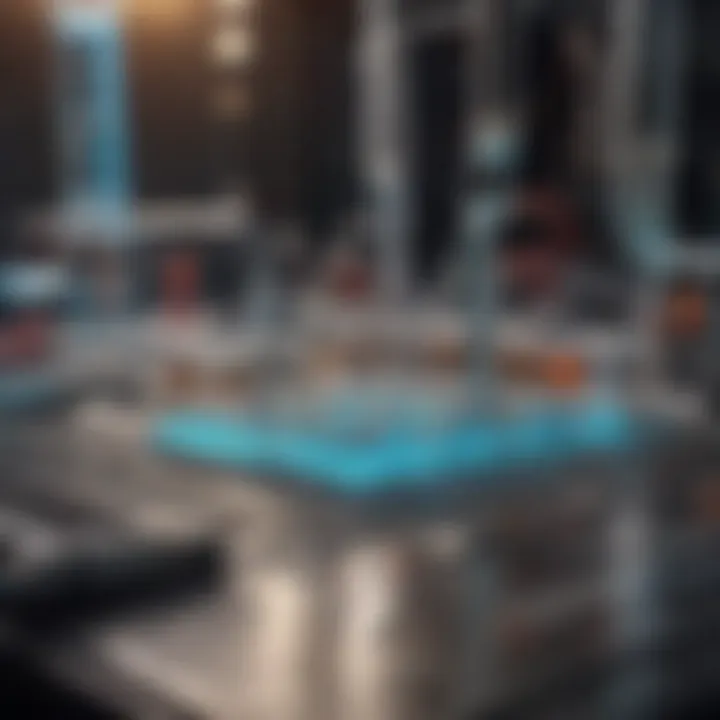
This fundamental understanding allows for the calculation of unknown values in electric circuits, leading to the efficient design of devices ranging from simple resistors to complex integrated circuits.
Furthermore, analyzing circuits through tools such as Thevenin’s and Norton’s theorems empowers engineers to simplify complex networks into manageable forms. This reduction aids in the development of more accurate and reliable systems, particularly in designing power supplies and amplification circuits used in audio electronics.
Integrating these principles of electronic flow and circuit theory into education and practice prepares students and professionals for innovative advancements. As we continue to explore the dictates of electronic flow in physics, we recognize its irreplaceable influence not only in theoretical constructs but in tangible, real-world technology.
Electronic Flow in Chemistry
The exploration of electronic flow in chemistry is crucial as it sheds light on how electrons behave and interact within chemical systems. Understanding electronic flow allows us to grasp the very essence of reactions and conductivity, paving the way for new innovations in both theoretical and practical fields. At its core, electronic flow links to more than just abstract concepts; it's about the lifeblood of chemical processes that sustain various forms of life and technology.
Redox Reactions
Redox reactions stand at the forefront of electronic flow, showcasing how electrons transfer during chemical changes. The term ‘redox’ derives from the combination of two processes: reduction and oxidation. In simple terms, reduction is the gain of electrons, while oxidation is the loss of electrons. Through this dance of electrons, substances undergo transformations that are vital in numerous scenarios, from energy production to metabolism in living organisms.
In a typical redox reaction, one reactant donates electrons — becoming oxidized — while another gains electrons, resulting in its reduction.
For example:
- In the oxidation of iron, iron loses electrons to oxygen, forming iron oxide.
- Conversely, in the reduction of copper ions, copper ions gain electrons to become solid copper.
These processes are not just limited to laboratory settings; they are fundamental to biological systems, particularly in enzymatic activities within cells, where electron flow is responsible for the transformation of substrates into products. Knowing how to control and utilize these reactions is what makes them a linchpin in fields like organic chemistry and biochemistry.
"Redox reactions can't be viewed in isolation. They are interconnected processes essential for understanding a breadth of chemical principles and applications."
Conductivity and Electrolytes
Shifting focus, conductivity and the role of electrolytes exemplify another dimension of electronic flow in chemistry. Conductivity refers to a substance's ability to conduct electric current, a property that hinges heavily on the presence of free-moving charged particles. Electrolytes, substances that dissociate into ions when dissolved in a solvent, are key in facilitating this conductivity.
Common examples include:
- Sodium chloride, which breaks apart into sodium and chloride ions when mixed with water.
- Potassium nitrate, another salt that dissociates to allow ions to flow freely.
Electrolytes create currents in solutions, contributing to various applications such as batteries, sensors, and even physiological functions like nerve impulse transmission in animals. When electrolytes are abundant, they enhance conductivity, which is pivotal in numerous chemical reactions, including those carried out in electrochemical cells, where the flow of electrons generates electric currents.
In indusry, understanding the properties of electrolytes can significantly impact the design of energy storage systems. Innovations in battery technology, such as lithium-ion batteries rely heavily on the efficient flow of ions, echoing the importance of electronic flow in chemistry throughout modern energy solutions.
In this respect, mastering the nuances of electronic flow in chemistry not only benefits theoretical learning but also equips researchers and engineers with the insight necessary to make strides in technological advancements.
Biological Implications of Electronic Flow
Understanding electronic flow in biological systems is crucial. It highlights how creatures sustain life at a cellular level and how energy conversion occurs. This section explores pivotal elements such as cellular level mechanisms and the electron transport chain, illustrating how electronic flow integrates within the fabric of living organisms.
Cellular Level Mechanisms
At the cellular level, electronic flow plays a crucial role in various biological processes. The movement of electrons enables crucial reactions that sustain cellular activities. Take, for example, the role of ion channels in neuronal signaling. Neurons transmit signals through action potentials, facilitated by the rapid influx and efflux of ions, resulting in electronic flow across the cell membrane.
The mechanisms at play involve major players like sodium (Na+) and potassium (K+) ions. The Na+/K+ ATPase pump utilizes ATP to maintain the concentration gradients across the neuronal membrane, creating conditions ripe for electronic flow. When a neuron fires, voltage-gated sodium channels open, allowing sodium ions to rush in. This influx leads to a rapid depolarization of the membrane, a pivotal step for transmitting signals.
Moreover, in muscle cells, electronic flow is the heartbeat of contraction. The electrical signals trigger the release of calcium ions from the sarcoplasmic reticulum, which facilitates muscle contraction. Without these electronic interactions, muscle movements would simply be impossible.
Electron Transport Chain
Another fundamental aspect of electronic flow in biology is the electron transport chain (ETC). This process, primarily taking place in the mitochondria, is crucial for cellular respiration. Through a series of proteins embedded in the mitochondrial membrane, electrons derived from nutrients are transferred. This transfer releases energy, which is harnessed to generate ATP, the energy currency of the cell.
Here’s how it unfolds:
- Electron Carrier Molecules: NADH and FAD carry high-energy electrons to the ETC, derived from the breakdown of glucose in earlier stages of metabolism.
- Complexes within Mitochondrial Membrane: As electrons move through these complexes, such as NADH dehydrogenase, cytochrome bc1 complex, and cytochrome c oxidase, they help pump protons (H+) into the intermembrane space, creating a proton gradient.
- ATP Synthesis: The protons flow back into the mitochondrial matrix through ATP synthase, driving the synthesis of ATP from ADP and inorganic phosphate.
This chain of events showcases the beauty of electronic flow in sustaining life. The synergy of chemical reactions and electron flow is not just pivotal but essential in supporting complex life forms. Additionally, understanding this chain can illuminate potential avenues for interventions in metabolic disorders where energy production falters.
"The fundamental interplay of electronic flow and biochemical processes underlies the essence of life itself."
As such, the biological implications of electronic flow extend far beyond mere mathematical concepts. They intertwine with life processes in ways that touch upon energy production, signaling, and an organism's overall functionality.
Technological Applications
In today's rapidly advancing world, the application of electronic flow plays a vital role in various technologies that form the backbone of modern life. Understanding these applications not only sheds light on how devices operate but also opens doors for future innovations. Electronic flow serves as the bedrock upon which countless advancements stand, impacting everything from consumer electronics to energy systems.
Semiconductor Devices
Semiconductors form the cornerstone of modern electronic devices. These materials, which fall between conductors and insulators in their electrical properties, have transformed how we think about and utilize electricity. The principles of electronic flow are intricately woven into the operation of semiconductor devices.
- Transistors: Transistors are the building blocks of microchips, enabling amplification and switching functions essential for creating complex circuits. At their core, they rely on the movement of electrons and holes—essentially the absence of electrons—facilitating the flow of electronic signals.
- Diodes: Diodes allow current to flow in one direction while blocking it in the other, a fundamental feature that makes them crucial for converting alternating current (AC) to direct current (DC). They are employed in everything from power supplies to signal modulation in communication systems.
- Integrated Circuits: The culmination of semiconductor technology, integrated circuits combine thousands of tiny components into a single chip. The efficient flow of electricity through these components revolutionizes computing and telecommunications, leading to devices that fit in our pockets yet possess the power of early supercomputers.
Semiconductor devices highlight the importance of controlling electronic flow. By managing how and where electrons move, engineers are paving the way for faster, smaller, and more efficient technologies.
Energy Harvesting Technologies
As the world becomes more energy-conscious, the ability to harness energy from the environment is becoming increasingly relevant. Energy harvesting technologies, grounded in the principles of electronic flow, allow us to capture energy that would otherwise be wasted and convert it into usable power.
- Photovoltaic Cells: These devices convert sunlight directly into electricity through the photovoltaic effect. The electronic flow induced by sunlight in semiconductor materials leads to the generation of electrical power. This technology represents a significant step towards sustainability.
- Thermoelectric Generators: These systems convert temperature differences into electrical energy. By exploiting electronic flow due to thermal gradients, they can turn wasted heat from industrial processes into supplementary power.
- Piezoelectric Devices: Piezoelectric materials generate electric charge in response to applied mechanical stress. These devices can be integrated into everyday environments, such as on roads and sidewalks, to create electricity from the pressure of footsteps or vehicles.
This quest to harness energy from our surroundings not only addresses energy demand but also reduces our carbon footprint, marking a significant stride toward sustainability.
Measuring Electronic Flow
Understanding electronic flow requires not only theoretical underpinnings but also practical measurement techniques. Measuring electronic flow is a pivotal aspect, allowing researchers and practitioners to quantify how charge moves through conductors and semiconductors alike. By grasping these measurements, one can unleash insights into the functionalities of modern electronics, the efficiency of energy systems, and even biological processes at the cellular level.
The importance of this topic lies in its ability to bridge theory with practice. Accurate measurements are essential for developing new technologies, assessing existing systems, and even fault diagnosis in circuits. Furthermore, in research, substantial proof often hinges upon the data arising from these measurements. Without precise tools and techniques to measure electronic flow, the confidence in outcomes diminishes.
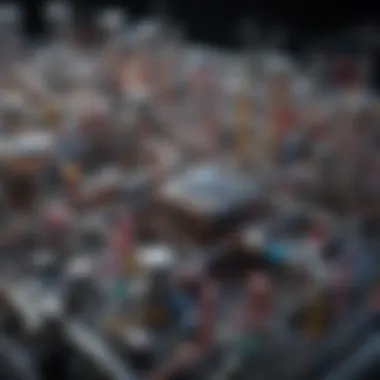
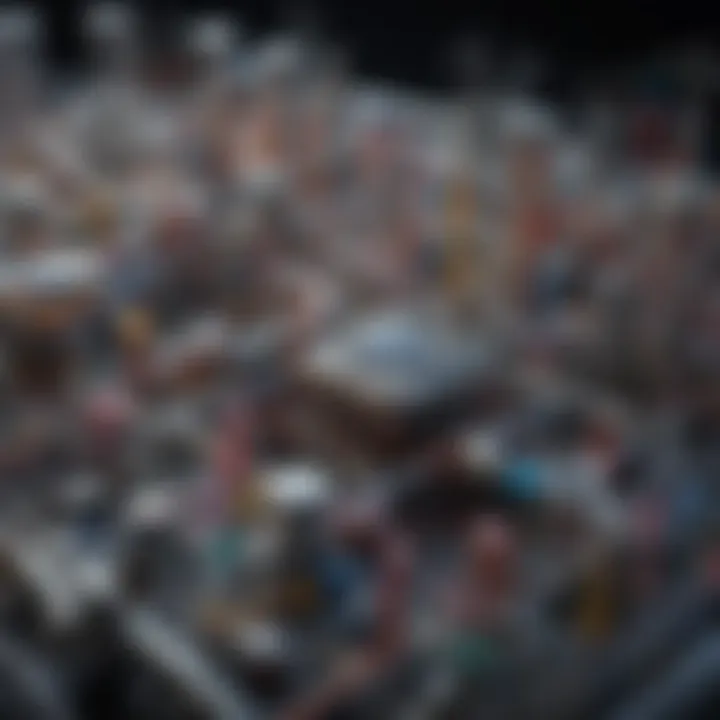
Instrumentation Techniques
In measuring electronic flow, instrumentation techniques play a critical role. Various devices are available to capture these readings, each with its unique characteristics and applications. Some common instrumentation techniques include:
- Oscilloscopes: These devices allow observation of the waveform of electronic signals, displaying voltage changes over time. They are particularly useful for analyzing complex signals and transient responses in circuits.
- Multimeters: These are versatile tools that can measure voltage, current, and resistance. Though simple, they provide essential insights into electronic flow in circuits and are often the first line of diagnosis for faults.
- Four-terminal sensing: This technique reduces the impact of lead resistance, improving measurement accuracy, especially in low-resistance measurements, which is critical in fields such as semiconductor testing.
It’s crucial to choose the right tool for the right context. A tailored approach provides a more precise measurement suited to the specific characteristics of the material or the nature of the experiment.
Data Interpretation and Analysis
Once the electronic flow is measured, the next formidable challenge is interpreting the data. Simply gathering numbers doesn’t give the full picture; understanding what those numbers mean is essential. Here, statistical analysis and simulations often come into play.
- Statistical Methods: Analyzing distributions and variations in measured flow can give insights into stability and performance. For instance, looking at deviations from expected values can indicate issues in system design or operation.
- Simulation Models: Coupling measurements with computer simulations allows researchers to validate their theories and assumptions. Through simulations, one can model expected behaviors based on measured data, thus revealing underlying trends that might not be immediately evident from the raw data alone.
Moreover, visualization techniques are integral in data interpretation. Graphing data effectively can highlight patterns or anomalies, paving the way for deeper analytical insights. Proper interpretation helps not only in reporting findings but also in making informed decisions based on those findings.
Rather than viewing measurement and analysis as mere steps, think of them as interconnected processes that enrich your overall understanding of electronic flow.
Achieving mastery in these areas is particularly vital for students and professionals alike, as they form the foundation for experiments and applications across various scientific disciplines.
Challenges in Understanding Electronic Flow
The investigation of electronic flow presents several challenges that researchers and educators must navigate. The complexities inherent in this topic are not merely academic curiosities; they have significant implications for technological advancements and the development of new materials. Understanding these challenges helps clarify why the study of electronic flow is so crucial. Here, we will focus on two primary obstacles: experimental limitations and complex interactions in materials.
Experimental Limitations
Effective study of electronic flow greatly depends on the precision of experimental methods available to scientists. However, limitations in measuring instruments can hinder the accurate assessment of electronic properties.
- Sensitivity of Equipment: Measuring minute electronic flows requires instruments with high accuracy. Devices like oscilloscopes and electron beam equipment can be expensive and challenging to manage.
- Environmental Control: Electronic behavior can change under different environmental conditions, such as temperature and pressure. Replicating the exact conditions necessary for consistent measurements is often impractical in laboratory settings.
- Sample Quality: The materials themselves can be flawed due to impurities or defects, leading to erroneous findings. Even slight variations can skew results, making it difficult to draw generalized conclusions.
Based on these factors, researchers often find themselves facing a double-edged sword—while advancements in technology continue to push boundaries, new limitations in experimental procedures arise constantly.
Complex Interactions in Materials
The intricate makeup of materials adds an additional layer of complexity to our understanding of electronic flow. The interactions between electrons and their surrounding environments are not straightforward and can differ vastly depending on the material in question.
- Quantum Effects: At the atomic level, electronic behavior can be influenced by quantum mechanics. For instance, phenomena such as tunneling and superposition complicate our grasp of electron flow. This leads to challenging questions about how to model these behaviors accurately within established theories.
- Material Heterogeneities: Different materials exhibit varying conductive characteristics. In composites, for example, layers of dissimilar materials may interact unpredictably, resulting in unique electronic behavior that defies traditional understandings.
- Thermal and Mechanical Stresses: The introduction of mechanical or thermal stress can cause unexpected disruptions in electronic flow; factors that are often overlooked in theoretical models. This has a direct impact on the reliability of devices that rely on specific electronic parameters.
In summary, the challenges in understanding electronic flow are rooted in both the experimental conditions researchers must endure and the complex interactions that occur within materials. As we strive to unravel these puzzles, the field continues to evolve, leading to deeper insights and potential breakthroughs in technology.
Recent Developments in Electronic Flow Research
The field of electronic flow research has recently seen remarkable advancements that have a profound impact on various scientific disciplines. These developments not only enhance our understanding of electronic flow but also bridge gaps across physics, chemistry, and biology. The integration of fresh methodologies and technologies has propelled this area to new heights, making it a critical subject for scholars, researchers, and industry professionals alike.
Innovations in Material Science
Material science has witnessed a slew of innovations aimed at improving our grasp of electronic flow. One notable advancement is the creation of novel materials that significantly enhance electrical conductivity. Researchers are exploring graphene and carbon nanotubes, which possess extraordinary conductive properties. These materials have opened avenues for their application in electronics and energy systems.
- Graphene: This single layer of carbon atoms has become a buzzword in the realm of material science due to its incredible strength and conductivity. It allows for faster electron movement, which can dramatically increase the efficiency of electronic devices.
- Carbon Nanotubes: These cylindrical structures also exhibit remarkable electrical properties. Their potential use in creating flexible electronics and enhancing solar cell efficiency is particularly promising.
Moreover, the use of organic materials in electronics is gaining traction. Organic semiconductors offer flexibility and a lower environmental footprint compared to traditional inorganic materials. This shift to organic materials reflects a broader trend towards sustainability in electronic flow research.
Emerging Theories and Models
As innovations continue, so do the theoretical frameworks that seek to explain the behavior of electronic flow in complex systems. New theories being proposed challenge traditional models, presenting a more nuanced view of electron behavior and interactions within various materials.
- Quantum Tunneling: This phenomenon, where electrons pass through barriers, is receiving renewed attention. Understanding quantum tunneling can lead to breakthroughs in nanoscale electronic applications and improve the design of memory devices.
- Non-Equilibrium Thermodynamics: This emerging model considers how electronic flow operates outside of equilibrium states, offering insights into how systems evolve under varying conditions. It is particularly relevant in the study of biological systems where electron flow can be driven by gradients and fluxes.
The intersection of these new theories with experimental findings is paving the way toward a more comprehensive comprehension of electronic flow, supporting interdisciplinary collaborations that are essential for future discoveries.
"Innovations in material science and evolving theories are reshaping our understanding of electronic flow, making it a vibrant field of study that holds promise for practical applications and fundamental science."
As the foundations of electronic flow continue to evolve, future research must focus on harnessing these developments to solve real-world problems, ranging from advanced electronics to sustainable energy solutions.
Interdisciplinary Connections
Interdisciplinary connections form the bedrock for innovative solutions in understanding electronic flow. The synergy between various scientific domains enriches research, leading to a holistic grasp of complex phenomena. The interplay between physics, biology, chemistry, and engineering creates avenues for collaborative exploration, enhancing not only academic research but also practical applications that benefit society at large.
By bridging gaps between distinct fields, researchers can tackle multifaceted issues that demand a comprehensive understanding of electronic flow. For instance, physicists working alongside biologists can study the implications of electron transport in cell signaling pathways. This cross-pollination of ideas often leads to groundbreaking insights that would remain unexplored in isolation.
Moreover, the importance of these connections cannot be overstated in terms of educational strategies. When students see how electronic flow integrates across disciplines, it fosters a more significant interest in science and promotes a deeper understanding of its relevance in real-world contexts.
Ultimately, these interdisciplinary collaborations ensure a richer understanding of the fundamental aspects of electronic flow, benefitting researchers, educators, and students alike.
Physics and Biology Intersection
The intersection of physics and biology presents an intriguing landscape for exploring electronic flow. At this crossroads, theories of charge movement have been applied to unravel complex biological processes, especially at the cellular level. The principles of electromagnetism, for instance, are critical in studying how nerve impulses travel along neurons due to the conduction of electrical signals.
In numerous physiological systems, the flow of electrons is key. When it comes to muscle contractions, electrical impulses trigger a cascade of biochemical responses that enable movement. Thanks to advances in imaging technologies, the visualization of electron dynamics in living organisms has become possible. This understanding can lead to inventive therapies for diseases related to electrical signaling errors, like epilepsy.
Researchers have found that manipulating electronic flow could provide treatments that target specific pathways, resulting in better health outcomes.
"The blending of physics principles into biological applications not only enhances scientific inquiry but also encourages innovative thinking that can lead to significant medical advancements."
Chemistry and Engineering Collaborations
Chemistry and engineering collaborations make for another powerful connection that amplifies the understanding of electronic flow. In chemical engineering, the study of conductivity in materials has paved the way for designing more efficient energy systems. A prime example is the development of advanced batteries and fuel cells, where optimizing electron transport directly impacts energy efficiency.
Furthermore, materials chemistry plays a vital role in creating semiconductors essential for electronic devices. An understanding of how electronic flow operates in these materials can lead to breakthroughs in developing new technology.
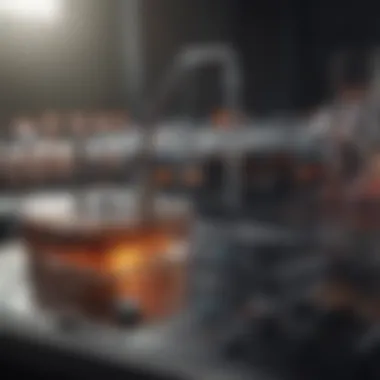
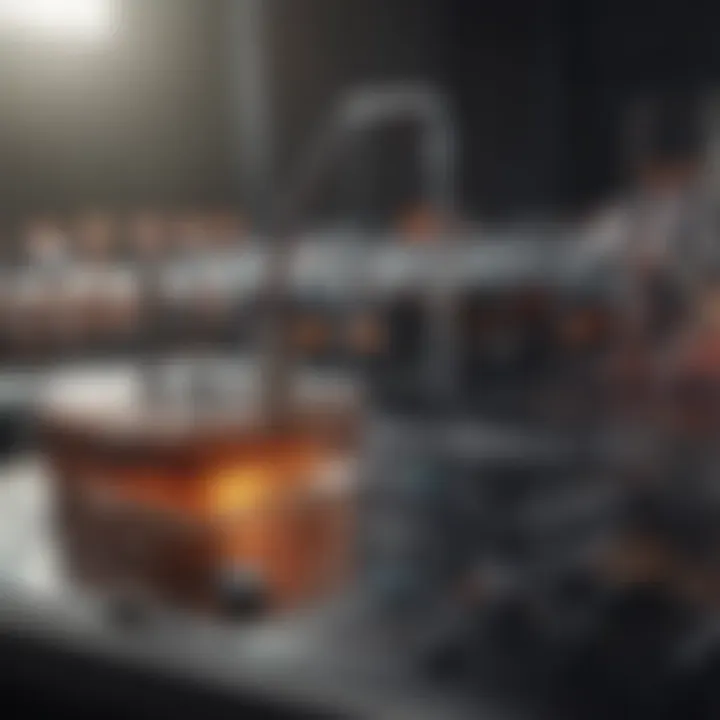
Likewise, engineers leverage principles from chemistry to invent novel sensors that detect specific chemical changes by monitoring electronic flow. These sensors can be pivotal in a range of industries, including environmental monitoring, healthcare diagnostics, and food safety.
By encouraging chemists and engineers to work side by side, we can push the boundaries of what electronic flow can achieve practically and effectively.
Educational Aspects of Electronic Flow
Understanding electronic flow is not just a niche interest for specialists in physics or chemistry; it serves as a foundational theme that connects multiple scientific disciplines. It is crucial for students and educators to grasp the relevance and implications of this topic for a well-rounded education. Incorporating electronic flow into the curriculum can enable better comprehension of complex scientific concepts, promote interdisciplinary learning, and cultivate critical thinking skills.
The integration of electronic flow into educational settings paves the way for innovative teaching and learning opportunities. In classrooms, students can engage with real-world applications such as energy systems or electronic devices, enhancing their overall educational experience. By showcasing how electronic flow underpins technology advancements—like solar cells or semiconductor devices—students can appreciate its significance beyond theoretical concepts.
Moreover, fostering discussions about electronic flow encourages students to consider ethical dimensions and the impact of technology on society. These discussions help cultivate responsible future scientists and informed citizens, aware of both the benefits and potential pitfalls of scientific advancements.
Curriculum Integration
When integrating electronic flow into school or university curriculums, the challenge lies in making abstract concepts accessible. Taking a comprehensive approach can transform how students perceive and understand electronic flow. There are multiple frameworks to consider:
- Hands-On Experiments: Lab activities using simple materials can illustrate principles of charge and current flow. For instance, building basic circuits can bring the topic to life, helping students visualize functions and results.
- Thematic Units: Linking electronic flow with broader concepts in physics and chemistry ensures that students can make connections. For example, studying electrochemistry alongside traditional chemistry topics can reinforce the understanding of redox reactions.
- Assessment Variety: Employing diverse assessment methods—research projects, group discussions, and practical demonstrations—can capture different learning styles, encouraging students to engage at multiple levels.
Equally vital is the need to adapt curriculums to reflect advancements in technology and research. Continuous updates to educational content allow students to stay abreast of recent developments, such as emerging theories in electronic flow, thus aligning classroom learning with current scientific discourse.
Teaching Methodologies
To convey the intricacies of electronic flow effectively, educators can adopt several teaching methodologies tailored to elevate student engagement and comprehension:
- Inquiry-Based Learning: This method invites students to pose questions and conduct investigations. For instance, letting them explore how varying material conductivity affects electronic flow in circuits stimulates critical thinking.
- Collaborative Learning: Encouraging group projects on topics like the practical applications of electronic flow in renewable energy sources fosters teamwork and communication skills while enhancing their understanding through peer learning.
- Flipped Classroom: Providing instructional content for students to explore independently and using class time for hands-on activities allows for deeper engagement with the material, optimizing classroom dynamics.
To ensure that students absorb these pivotal concepts effectively, it’s essential to employ methods that resonate with their experiences and interests. By tailoring educational practices around the students' context, educators can help demystify electronic flow, ultimately sparking passion and inquiry in the next generation of scientists.
Future Directions in Electronic Flow Research
As the quest to unravel the complexities of electronic flow continues, the future of research in this area promises to be both intriguing and vital. Understanding electronic flow is not merely an academic exercise; it holds the potential to influence technologies ranging from renewable energy sources to biomedical devices. The intersections of various disciplines ensure that new revelations can surface at any moment, igniting innovation and enhancing our lives.
Potential Breakthroughs
Well, if we look closely, the potential breakthroughs in this realm can reshape entire industries. One of the most bullish aspects is the evolution of materials exhibiting extraordinary conductive properties. Think of graphene, the supermaterial—its remarkable strength and conductivity can redefine how we think about electronic applications. Then consider quantum dots for enhanced solar cells, offering a path to make solar energy not just a feasible alternative but a leading source of power.
Moreover, researchers are eyeing molecular electronics, where single molecules toggle between conductive and insulating states depending on external stimuli. This presents a tantalizing glimpse into faster computing and more efficient data storage.
"The breakthroughs in electronic flow research could one day lead us to technologies we can't even fathom today."
Additionally, the advancements in bioelectronics suggest that merging biological systems with electronic circuits can revolutionize medical therapies, especially for diseases that currently have limited treatment options. Picture biosensors embedded in our bodies, analyzing chemical signals and alerting patients and doctors to health issues before they escalate.
Funding and Resource Allocation
Funding and resource allocation remain pivotal for catalyzing research into electronic flow. The reality is that significant financial support can determine the pace and success of investigatory projects. For instance, government grants and private investments can bridge the gap between theoretical research and practical application. Priority may be given to innovative projects that promise sustainability or major advancements in health technology.
Resource allocation should also include interdisciplinary collaboration. Scientists, engineers, and industry professionals need open channels of communication to share insights and speed up the research cycle. Consider that integrating knowledge from materials science and electronics can spark fresh ideas that single disciplines might overlook.
Clearly identifying priorities in research can maximize impact. Focusing on sustainable practices in electronic flow technologies not only addresses environmental concerns but also attracts interest from funding bodies that emphasize green innovations. When the public sees the potential benefits—like cleaner energy or more efficient electronic devices—they are more likely to support such initiatives.
In summary, future directions in electronic flow research are a fertile ground for discovery. Potential breakthroughs in novel materials and bioelectronics alongside strategic funding could unlock new possibilities, thereby cementing electronic flow's role in the advancement of modern science and technology.
Ethical Considerations
The concept of ethical considerations in the study of electronic flow is not merely an afterthought; rather, it serves as a cornerstone for advancing scientific inquiry and application. As researchers, educators, and students delve into the implications of electronic flow across various disciplines, they must tread thoughtfully. Ethical standards ensure that the pursuit of knowledge does not come at an unacceptable cost—be it environmental, social, or educational. The exploration of electronic flow engenders several specific considerations, including environmental impacts, responsible research practices, and the broader societal implications that result from introducing new technologies.
Environmental Impact
When we discuss the environmental impact of research centered around electronic flow, it introduces a myriad of complex factors. The production of electronic devices, which plays a pivotal role in harnessing electronic flow, often leads to hazardous waste and significant energy consumption. Notably, a significant environmental concern arises during the mining of raw materials like coltan, crucial in electronics manufacturing. This mining process can wreak havoc on local ecosystems, leading to soil erosion and habitat loss.
Moreover, the disposal of electronic waste (e-waste) represents a growing dilemma. E-waste frequently contains toxic substances, such as lead and mercury, that can leach into the environment, posing health risks to nearby communities and wildlife alike. Therefore, it is imperative for researchers and industries to adopt sustainable practices, from designing for recyclability to implementing waste-minimization strategies. This commitment to responsible environmental stewardship is not only ethically sound but also vital for ensuring the longevity of our planet.
"An ethical approach to electronic flow research not only protects our environment but also enhances the credibility and social relevance of scientific inquiry."
Responsible Research Practices
Responsible research practices encompass the ethical treatment of all elements involved in electronic flow studies, including subjects, materials, and data. In this rapidly evolving field, maintaining integrity is crucial. The significance of transparency in research cannot be overstated. Researchers must present their findings openly while addressing potential conflicts of interest. This principle applies as much in academic settings as in industrial research and development.
In the context of electronic flow, it is essential to ensure that all experimental methodologies are ethically aligned. This could mean that studies involving biological subjects—where electronic flow may influence cellular mechanisms—must prioritize welfare and minimize harm. Equally, when dealing with sensitive data, adherence to best practices in data management and privacy is non-negotiable. Researchers ought to ensure proper consent, especially when human subjects are involved, and maintain confidentiality with all participant data.
Moreover, collaboration across disciplines—bringing together physicists, chemists, biologists, and engineers—can foster an environment of ethical consideration that bolsters innovation while safeguarding against unethical practices. A culture of shared responsibility will not only enrich scientific outputs but also positions the community to address tough questions as our knowledge about electronic flow expands.
In summary, the ethical landscape surrounding electronic flow research is vast and multifaceted, invoking a need for a conscientious approach to both environmental sustainability and responsible research practices.
Finale and Summary
The exploration of electronic flow culminates in a recognition of its pervasive role across various scientific disciplines, signifying not just theoretical importance but also practical applications that resonate in our daily lives. From the intricate behavior of electrons in physics to their crucial roles in chemical reactions, understanding electronic flow becomes paramount. Every section of this article has aimed to shed light on distinct yet interconnected aspects of electronic flow, painting a cohesive picture of its wide-ranging implications.
As we reflect on the significance of this topic, it stands out that the conversation around electronic flow is not a mere academic pursuit. Instead, it carries with it the potential to drive innovations in technology, medicine, and environmental science. For instance, integrating electronic flow concepts into the design of semiconductor devices continues to push the boundaries of efficiency and functionality in electronics. Moreover, the implications in biological systems, such as how cellular mechanisms utilize ionic currents, are manifestations of electronic flow's profound implications.
"The understanding of electronic flow transcends disciplines, facilitating a dialogue between physics, chemistry, and biology."
Recapitulating Key Points
In revisiting the core concepts discussed throughout the article, a few key elements emerge:
- Foundation in Physics: The principles of electronic flow lay their roots in fundamental physics, where charge and current are understood through circuit theory, significantly contributing to modern electrical engineering.
- Chemical Interaction: The relationship between electronic flow and chemical processes, particularly in redox reactions, highlights how electrons drive essential reactions that sustain life.
- Biological Frameworks: In biology, electronic flow illustrates the cellular mechanisms crucial for energy production and metabolic functions, especially through pathways such as the electron transport chain.
- Technological Advances: The advancements in semiconductor technology derive directly from an enhanced understanding of electronic flow, impacting everything from consumer electronics to renewable energy solutions.
Implications for Future Research
Looking ahead, the exploration of electronic flow harbors numerous opportunities for future research:
- Material Innovations: Investigating new materials that can enhance electronic conductivity and efficiency presents a domain ripe for discovery, potentially resulting in reduced energy loss and improved device performance.
- Sustainable Applications: The integration of electronic flow principles into renewable energy systems, such as solar panels and batteries, could lead to significant environmental benefits. Fostering innovations in these areas aligns well with global sustainability goals.
- Interdisciplinary Collaborations: Future research can benefit enormously from the convergence of disciplines. Physicists collaborating with chemists and biologists can uncover novel insights into complex electronic behaviors, fostering a deeper understanding of life at a molecular level.
- Enhanced Educational Models: Developing effective teaching methodologies that integrate these findings will be essential in preparing the next generation of scientists and engineers to tackle challenges that stem from electronic flow complexities.
In essence, as we draw this exploration of electronic flow to a close, the significant breadth and depth of its impact cannot be overstated. Continued research and interdisciplinary collaboration are crucial for harnessing the full potential of electronic flow, ensuring it remains a cornerstone of scientific inquiry and technological advancement.