Fundamentals and Applications of X-Ray Diffraction
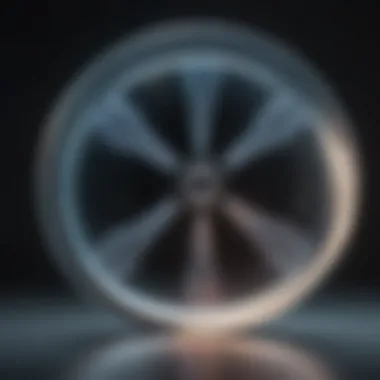
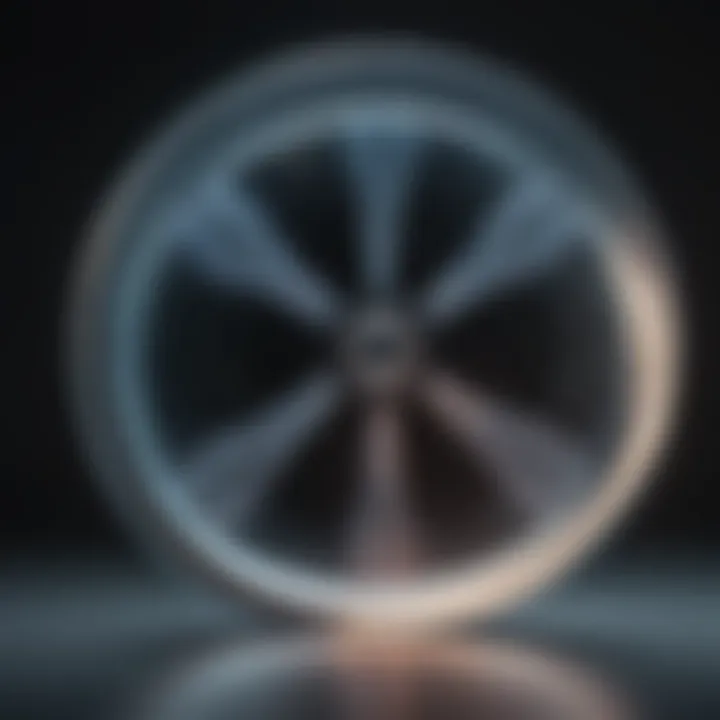
Intro
Understanding the arrangement of atoms in a crystal structure serves as a cornerstone for many scientific fields. Among the various techniques employed to discern these arrangements, single crystal X-ray diffraction stands out for its precision and depth of information it can provide. In essence, this method enables scientists to derive the three-dimensional arrangements of atoms within a crystalline solid, thus providing essential insights into the nature and behavior of materials.
In recent years, advancements in technology have made single crystal X-ray diffraction more accessible and efficient. The integration of sophisticated detectors and software has accelerated the process, paving the way for its broader application in industries ranging from pharmaceuticals to materials science.
Single crystal X-ray diffraction is not only pivotal in basic research but also plays a crucial role in applied science. The ability to accurately visualize molecular structures has far-reaching implications. In drug design, for instance, knowing the precise structure of a target protein can lead to the development of more effective pharmaceuticals. In materials science, understanding the crystal structure informants the design of materials with desired properties for various applications.
The following sections will delve into the complexities surrounding single crystal X-ray diffraction, discussing its underlying principles, methodology, and its far-reaching applications across different scientific spheres.
Preamble to Single Crystal X-Ray Diffraction
Single crystal X-ray diffraction stands as a cornerstone in the investigation of crystalline materials. Its essence lies in the ability to elucidate atomic arrangements within a solid. This method does not just scratch the surface; it penetrates to unveil intricate details that define the characteristics of various materials. Understanding this technique is crucial for researchers across multiple disciplines, including chemistry, biology, and materials science.
The benefits of single crystal X-ray diffraction are manifold. Primarily, it offers a non-destructive means to obtain precise structural information, which is vital in fields where the integrity of the sample must be maintained, like in drug discovery or the analysis of delicate biological materials. The data acquired provides a fundamental basis for further research and development, influencing everything from new drug formulations to advancements in nanotechnology.
Historical Background
Delving into the historical background of single crystal X-ray diffraction presents a rich narrative. The journey began in the early 20th century, with the work of Max von Laue, who won the Nobel Prize in Physics in 1914 for his groundbreaking experiments demonstrating that X-rays could be diffracted by crystals. This discovery opened up a new frontier in the field of crystallography, paving the way for many advancements.
As the decades progressed, various methods evolved, including the development of Fourier synthesis techniques in the 1930s, which facilitated the interpretation of diffraction patterns. By mid-century, the rise of sophisticated detectors and computers began to streamline data collection and analysis. What was once a slow, tedious process transformed quickly as technology progressed, allowing more scientists to harness the potential of this revolutionary technique.
Significance in Material Science
In material science, the significance of single crystal X-ray diffraction cannot be overstated. It serves as a vital tool to understand and design materials at the atomic level. The detailed insights gained through this technique can dictate the exploration and development of semiconductors, ceramics, and metals.
For instance, consider a new material undergoing synthesis. By applying single crystal X-ray diffraction, scientists can reveal its true structure and interpret how its unique arrangement may affect its properties. This understanding can significantly influence the material's performance in applications such as catalysis or electronic devices.
Additionally, the technique supports the quest for novel materials. Researchers seek out new compounds that display remarkable characteristics, and single crystal X-ray diffraction is crucial in confirming their structures. It aids in understanding phase transitions, stability, and potential for functionality, guiding the way for future innovations.
In summary, single crystal X-ray diffraction is more than just a method; it's a gateway into the world of atomic structures. Its history is rich and its relevance in material science is profound, making it an indispensable technique for today’s scientists.
Fundamental Principles
Understanding the fundamental principles of single crystal X-ray diffraction is central to this article, as these principles form the bedrock of how we analyze crystalline structures. Essentially, this section will elucidate the core tenets that allow scientists to extract vital insights into the arrangement of atoms within a crystal. These principles underscore not only the theoretical underpinnings of the technique but also its practical applications in diverse scientific fields.
X-Ray Interaction with Matter
When X-rays strike a crystal, they interact with the electrons surrounding the atoms. This interaction is pivotal; the extent to which X-rays are diffracted highly depends on the electron density of the crystal itself. Imagine shining a flashlight on a mirror; the angle at which the light reflects can change based on the surface and material properties. Similarly, X-rays will scatter at different angles depending on the atomic arrangement within the crystal lattice.
- Absorption: X-rays may be absorbed, depending on the material. The atomic numbers and electron configurations of the atoms in the crystal influence this absorption.
- Scattering: This can be coherent, elastic scattering resulting in diffraction patterns, while incoherent scattering leads to loss of information, which we want to avoid.
Understanding these interactions allows researchers to optimize experimental conditions, ultimately guiding better data interpretation.
Bragg's Law
Bragg's Law is a cornerstone concept in X-ray diffraction, capturing the relationship between the wavelength of the incident X-rays and the angles at which they are diffracted. This law posits that constructive interference occurs at specific angles when the path difference between rays scattered from consecutive lattice planes is an integral multiple of the wavelength:
nλ = 2d sin θ
Here, n represents the order of the diffracted beam, λ is the X-ray wavelength, d is the distance between atomic layers in a crystal, and θ is the angle of incidence. The simplicity of Bragg's Law doesn't diminish its significance; this equation lays the groundwork for calculating the spacing between lattice planes, a critical step in structural determination.
Reciprocal Space and Lattice Analysis
The concept of reciprocal space extends our understanding of crystal structures even further. In reciprocal space, every point corresponds to a set of lattice planes in the real space of the crystal. This mathematical construction allows for the visualization of the diffraction pattern as a series of spots, or reflections, which correlate with the periodicity of the underlying crystal structure.
- Lattice Vectors: These vectors define the periodicity in real space and are transformed into reciprocal vectors for analysis.
- Miller Indices: These indices categorize lattice planes and their corresponding diffraction peaks, simplifying the process of identifying and analyzing crystal structures.
By delving into reciprocal space, researchers can better infer how various parameters—like temperature or impurities—can change the structural characteristics of a material. It’s like flipping a switch, providing another perspective on the same fundamental information.
In summary, a solid grasp of these fundamental principles is vital for anyone looking to leverage single crystal X-ray diffraction in their research. The interplay between X-rays and matter, articulated through Bragg's Law and the framework of reciprocal space, provides a rich tapestry of insights into the atomic world, leading to myriad applications across scientific disciplines.
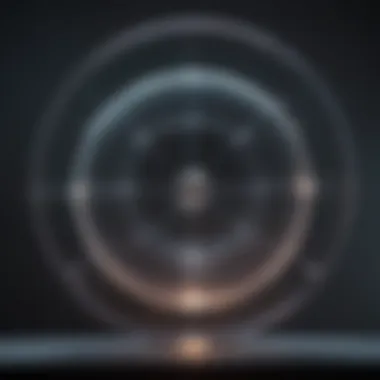
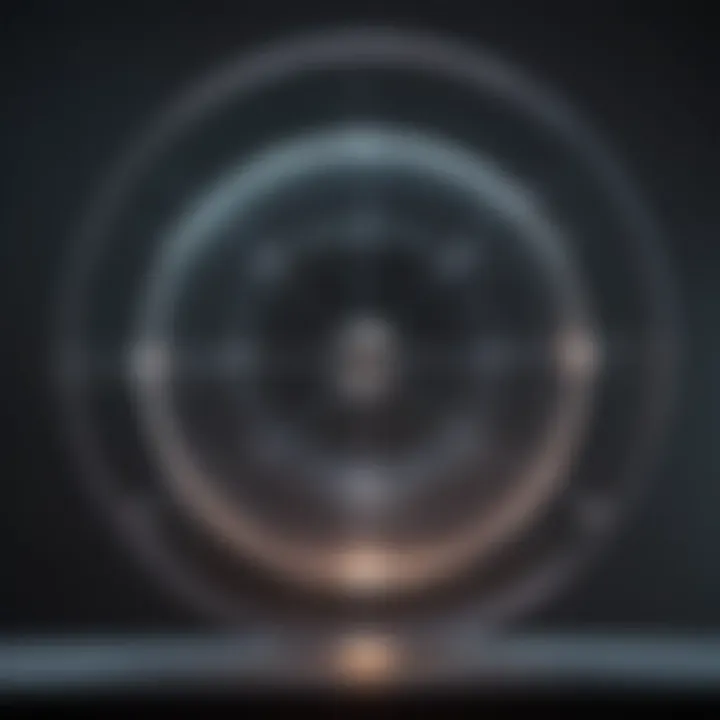
Experimental Setup
Understanding the experimental setup is pivotal in the field of single crystal X-ray diffraction. This section sheds light on the key elements that constitute the setup, where meticulous attention to detail directly affects the quality and accuracy of the obtained data. A solid experimental foundation not only enhances the resolution of the structural insights but also reduces the likelihood of errors during data analysis.
Sample Preparation Techniques
The journey of single crystal X-ray diffraction begins with sample preparation. This step often proves to be one of the most challenging aspects of the entire process. A good crystal is essential for quality diffraction data; hence, the methods of crystal growth and refinement are critical.
Common techniques used include:
- Vapor diffusion: A classic method where solvents evaporate slowly from a solution, promoting crystal growth. This requires patience and careful environmental control.
- Solvent evaporation: This faster technique involves allowing the solvent to evaporate from a saturated solution, where conditions must be right to avoid too rapid an evaporation that could yield poor-quality crystals.
- Melt crystallization: In situations where the compound has a melting point, melting and cooling the liquid can result in good crystal formation, but this requires high purity starting materials.
Each of these techniques is tailored according to the properties of the target compound and its solubility parameters. Apart from morphology, attributes such as size and uniformity greatly influence diffraction quality, thus making careful optimization necessary.
X-Ray Source and Detectors
Next up in the experimental setup is the X-ray source and detectors. Selecting the right x-ray source is about precision and efficacy. Typically, two main types of X-ray sources are considered: sealed tube sources and synchrotron radiation. Sealed tube sources are accessible and sufficient for many studies, while synchrotron offers significantly higher brightness and allows for faster data collection. This is particularly advantageous when working with delicate or transient samples.
The choice of detector is equally essential. Charge-coupled devices (CCDs) and image plates have become popular due to their sensitivity and efficiency. These detectors collect scattered X-rays and convert them into electronic signals, which can then be processed into useful diffraction patterns. This stage plays a crucial role in ensuring that the data obtained are of high quality and suitable for the subsequent analysis.
Data Collection Strategies
Finally, we dive into data collection strategies. Constructing an effective protocol for collecting diffraction data is essential for maximizing the quality of the dataset. Strategies often vary depending on the nature of the crystallized sample and the desired outcomes in terms of resolution and completeness.
- Rotational data collection is a common method where the crystal is rotated systematically, allowing for a comprehensive angular range of the diffraction pattern to be gathered.
- Continuous rotation can be employed for fast data acquisition, particularly useful for small crystals or samples that can potentially degrade upon exposure.
- Multi-wavelength anomalous dispersion (MAD) is a specialized technique used to enhance signal from specific atoms in the crystal, essential in solving the phase problem in certain contexts.
A well-designed data collection strategy can significantly reduce the time spent on data analysis and improve the reliability of results, leading to better quality manuscripts and advancements in research.
Data Processing and Analysis
Data processing and analysis form the backbone of single crystal X-ray diffraction. After the crucial steps of data collection, the challenge arises in interpreting the mountains of data generated. This aspect of the work is not merely an add-on to the experimental phase; it is fundamentally entwined with the accuracy and success of the findings. The complexities in crystallography necessitate that data be meticulously processed to yield reliable structural information. This is where the magic happens, turning raw readings into meaningful insights into molecular arrangements.
Integration of Diffraction Data
The first step in data processing is the integration of diffraction data. Here, one converts individual intensity measurements from the detector into a comprehensive dataset that represents the crystal structure. Each exposure yields a pattern that reflects how the X-rays interact with the crystal lattice. By applying precise algorithms, researchers aggregate these patterns, facilitating a coherent picture of atomic arrangements.
Utilizing software tools like MOSFLM or XDS, the datasets undergo corrections for various factors, including background noise and sample absorption. Importantly, this stage is critical as it helps in managing the differences that arise from potentially imperfect data captures. The success at this juncture lays the groundwork for subsequent analysis phases, promoting a clearer understanding of how molecules are arranged in three-dimensional spaces.
Phase Problem and Solutions
Addressing the phase problem is a significant hurdle in single crystal diffraction. This dilemma arises because X-ray diffraction provides only amplitude information, missing the critical phase. Without these phases, researchers face a brick wall in reconstructing the electron density map. Tackling this issue often requires clever methodologies.
Common strategies include:
- Direct Methods: These statistical approaches leverage the intensity data to estimate phases directly.
- MIR (Multiple Isomorphous Replacement): A technique that involves using heavy atom derivatives to ascertain phase information.
- SAD (Single-wavelength Anomalous Dispersion): This method exploits the unique absorption characteristics of certain elements in a structure to derive phase information.
Success in solving the phase problem can dramatically enhance structural resolution, enabling insights into molecular behavior crucial for fields like drug design and materials science.
"Solving the phase problem in X-ray crystallography often feels like finding a needle in a haystack —yet when solved, it opens doors to understanding the very fabric of materials and biological systems."
Refinement Techniques
With a reliable electron density map in place, the next step is refinement. This process aims to enhance the accuracy of the model by adjusting parameters to minimize the difference between observed diffraction patterns and those calculated from the structural model.
Common refinement techniques include:
- Least Squares Refinement: Here, deviations between observed and calculated data are minimized, giving a balanced view of the model's reliability.
- Simulated Annealing: This approach allows the model to escape local minima within a potential energy landscape, facilitating a more comprehensive search for the optimal structure.
- B-factor Refinement: Impacts the thermal motion of atoms in the crystal, it is essential to ensure that the model reflects the reality of the crystal’s dynamism.
Throughout this stage, the use of software such as CNS or Refmac allows for iterative improvement, where each round of refinement grounds the model more firmly in observed reality. The end goal is to reach a model that provides a trustworthy representation of the crystal structure, ready for interpretation.
By transparently addressing these intricate processes, the role of data processing and analysis becomes starkly clear, underscoring its essential contribution to the field of single crystal X-ray diffraction.
Applications of Single Crystal X-Ray Diffraction
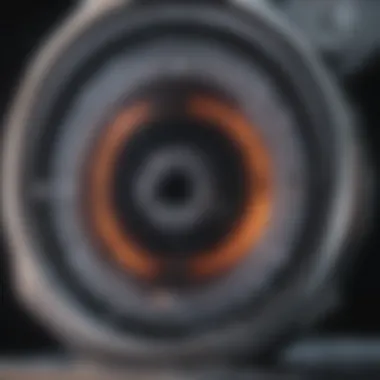
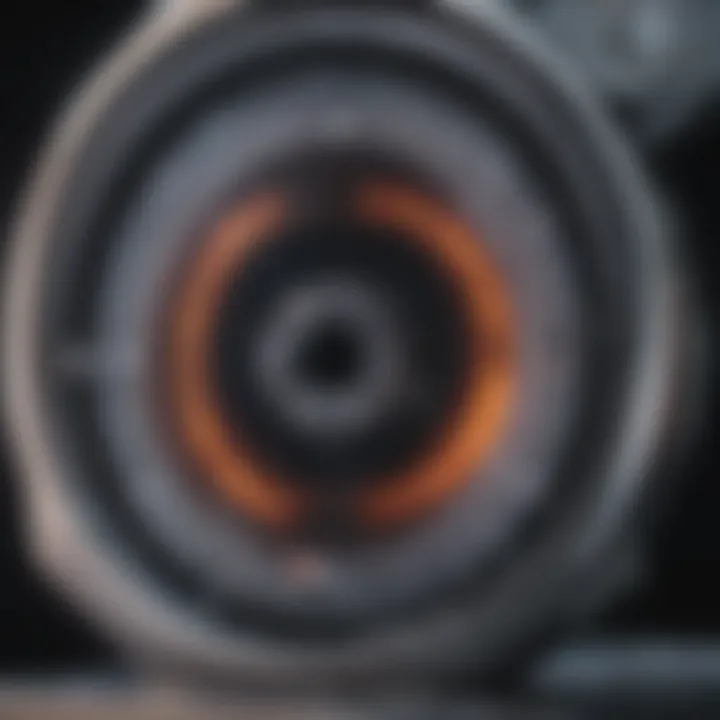
Single crystal X-ray diffraction is a cornerstone of various scientific fields. Its applications reach far beyond mere structural elucidation. This method has become essential in structural biology, materials science, and the pharmaceutical industry. In each of these areas, it helps unravel the complexities of matter on an atomic level, providing a deeper understanding that drives innovation and discovery.
Structural Biology
Protein Crystallography
Protein crystallography stands as a vital tool in the realm of structural biology. It allows scientists to decipher the three-dimensional structures of proteins at atomic resolution, unveiling their functional mechanisms. The key characteristic of protein crystallography is its ability to directly correlate structure with function. This correspondence is critical because subtle changes in structure can lead to profound effects on the biological function of proteins.
What makes protein crystallography a favorable choice is its efficiency in providing atomic-level insight that other techniques might struggle to achieve. For instance, circumventing the limitations of lower-resolution methods is crucial for drug discovery, where understanding the target structure is paramount. However, it’s worth noting that the technique requires high-quality crystals which can be finicky to grow. A limitation often faced is the time-intensive nature of crystallization processes.
Enzyme Mechanisms
Enzyme mechanisms are best studied through the lens of single crystal X-ray diffraction. Understanding how enzymes catalyze reactions can be profoundly insightful for biotechnology and medicine. The technique reveals detailed enzyme structures during different stages of catalysis, which is paramount for designing inhibitors or enhancers.
The defining characteristic of studying enzyme mechanisms via this method is the capacity to visualize transient states before and after catalysis. This is particularly beneficial because it leads to potential breakthroughs in drug design, where such insights can direct the development of more effective pharmaceutical agents. A unique feature of this approach involves capturing snapshots of enzyme-substrate interactions, although it may involve challenges related to achieving suitable crystallization conditions for the transient states.
Materials Science
Characterization of New Materials
In the materials science domain, characterizing new materials is a critical application of single crystal X-ray diffraction. The technique excels in determining the atomic arrangements in novel materials, paving the way for the discovery of new properties and potential uses. The key attribute here is the incredible resolution that allows scientists to correlate material properties directly with their crystal structure, a factor that can greatly influence electronic, magnetic, and optical characteristics.
This application is widely appreciated because it not only aids in the development of advanced materials but also helps researchers understand fundamental phenomena in crystallography. However, obtaining high-quality single crystals of new materials can be a challenging endeavor, often requiring meticulous work and creativity in synthesis.
Investigating Properties of Crystals
Investigating the properties of crystals through single crystal X-ray diffraction provides essential insights into their interactions and behaviors. This aspect allows for a detailed look into how different crystals interact within various environments, crucial for applications in electronics, optics, and photonics. A significant characteristic of this application is the ability to determine how structural variations influence functional characteristics, thereby guiding the design of custom materials.
Unique features of this method include its precision, but also its limitation in the sense that not all properties can be entirely explored through purely crystallographic data. Additional techniques may still be necessary to comprehensively understand dynamic behaviors or electronic properties.
Pharmaceutical Applications
Drug Design and Development
In the realm of pharmaceuticals, drug design and development benefit immensely from single crystal X-ray diffraction. It serves as a critical bridge between molecular design and biologically active compounds. The main draw of using this technique is its unparalleled ability to visualize drug-target interactions at an atomic level, which can inform the design of more effective drugs.
A unique feature here is the capability to refine lead compounds through iterative cycles of crystallization and structure determination, which is beneficial for optimizing drug efficacy and specificity. However, high-quality single crystal growth can often be time-consuming, posing a challenge.
Understanding Drug-Receptor Interactions
Understanding drug-receptor interactions is fundamental in developing effective therapies. Single crystal X-ray diffraction sheds light on how drugs bind to their targets, providing essential data for predicting drug behavior. The key characteristic is the capacity to elucidate binding modes, which informs how slight modifications to a drug molecule can enhance or diminish its activity.
This area is celebrated for its detailed structural insights, yet it can also face limitations when dealing with complex biological systems or transient interactions that may evade static crystallography. Integration with other analytical methods may be required to address these challenges.
Challenges and Limitations
The realm of single crystal X-ray diffraction is indeed fascinating, yet it is not without its hurdles. Understanding these challenges is paramount for students, researchers, and professionals seeking to navigate this technologically intense landscape. The outcomes of structural analysis are only as good as the data gathered, and any hurdles faced during the process can lead to inaccuracies that ripple through further studies. The significance of addressing these challenges cannot be overstated, as it directly influences the integrity of scientific discoveries in various fields.
Crystallization Difficulties
Crystallization serves as the foundation for single crystal X-ray diffraction work. However, achieving high-quality crystals can sometimes feel like chasing shadows. The chemistry and physical conditions required to grow suitable single crystals may vary widely among different materials. Factors that influence crystal growth include temperature, solvent choice, and concentration of the reagents. Moreover, impurities can easily interfere, complicating the intended process.
Consider the case of inorganic complexes. These often require specific environments and conditions for crystallization, making it a painstaking trial-and-error affair.
Some common issues in this domain include:
- Nucleation: The first step in crystal formation is crucial. If not controlled, too many nucleation sites can form simultaneously, leading to small, poorly formed crystals.
- Solubility limitations: Certain materials simply don’t want to dissolve well, hindering any attempts to initiate crystallization.
- Time constraints: Some crystals might take weeks, or even months, to grow. For fast-paced research settings, this can be a major hindrance.
These crystallization issues lead to a splash of frustration in the lab. Researchers unraveling new compounds or investigating novel materials often face the reality that good data can only come from good crystals. As such, the aim is to refine these methods continuously, seeking new techniques to improve crystallization outcomes.
Data Quality Issues

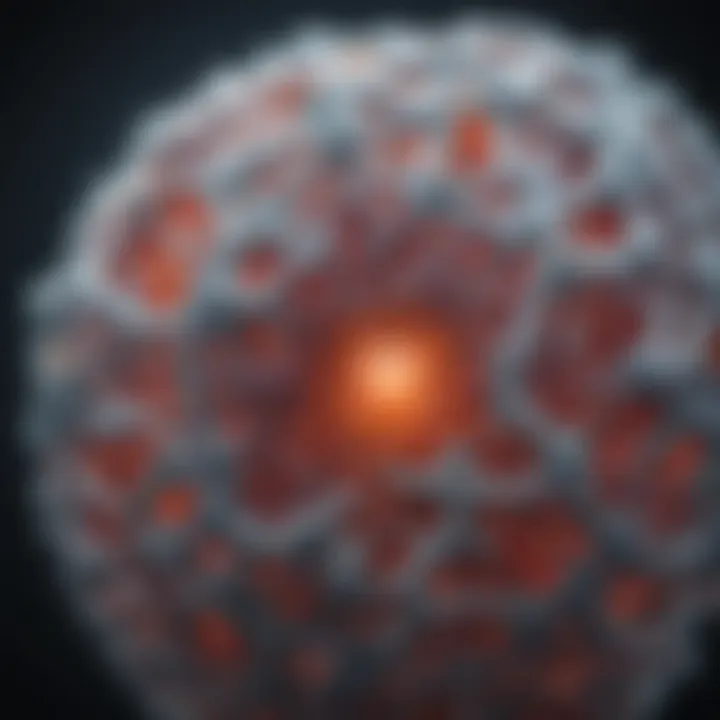
Once you have managed the delicate task of crystal growth, a new set of challenges emerges as focus shifts to data quality. Even the most perfectly grown crystals can yield unsatisfactory data if not approached correctly.
Inadequate data quality can stem from various sources, specifically:
- Instrumental imperfections: The equipment, no matter how cutting-edge, can experience calibration issues or might not be well-suited to particular applications.
- Measurement errors: Inaccurate alignment or positioning during the diffraction data collection can lead to flawed results. This is critical because even minor variations can substantially impact the study.
- Noise: Background noise during data collection may obscure vital signals from the crystal, complicating interpretation.
Data quality ultimately determines the reliability of structural information garnered through single crystal X-ray diffraction. As the mantra goes, “garbage in, garbage out.” Insufficient attention to detail in data acquisition can mean reconstructing models becomes equivalent to building sandcastles at tide's edge—all efforts washed away by inaccuracies.
Therefore, to improve outcomes in the face of these limitations, researchers need to invest time in improving their experimental setups and practices. Whether that means upgrading instruments, refining alignment techniques, or developing new algorithms for data analysis, the path to increased data integrity is critical.
"In the world of science, every challenge can turn into an opportunity for innovation."
Navigating these challenges and limitations may feels like walking a tightrope, but with persistence, ingenuity, and collaboration, the scientific community can overcome the hurdles that single crystal X-ray diffraction presents.
Future Trends in Single Crystal X-Ray Diffraction
The realm of single crystal X-ray diffraction (XRD) is poised for significant evolution. With ongoing research and innovation, researchers are bound to discover enhanced techniques and approaches that will improve accuracy, efficiency, and versatility in structural analysis. Keeping eyes on future trends in this area is not merely of academic interest; it holds implications for various fields, from material science to drug discovery. Understanding these evolving methodologies can help scientists extrapolate significant insights from crystallographic data, ultimately advancing both fundamental and applied science.
Technological Advancements
High-Resolution Techniques
When it comes to high-resolution techniques, one of its paramount contributions is the ability to achieve near-atomic detail in crystal structures. This approach emphasizes refining the X-ray beam and detectors, allowing for better resolution and clarity. A notable characteristic of high-resolution methods is their sensitivity to small changes in crystal position and disorder, making them invaluable in studies where precision is paramount. One such method, known as four-circle diffractometry, provides exceptional angular resolution and can even capture subtle vibrations of atoms, revealing dynamic behavior within the crystal lattice.
However, high-resolution techniques are not without drawbacks. They demand sophisticated equipment and can extend data collection times, impacting productivity in busy labs. Still, their depth of insight justifies their usage in studies where structural details are paramount.
Automated Data Collection
Turning to automated data collection, the significance is hard to overestimate. This advancement minimizes human error and enhances efficiency by allowing instruments to operate with minimal operator intervention. The key feature here is the seamless integration of automation with traditional data collection practices, which serves as a game-changer in overcoming time constraints typical in previous methods. It’s beneficial because it drastically shortens the data acquisition process while ensuring reproducibility.
Yet, while automation carries its perks, one should remain cautious. Higher dependence on automated systems can lead to overlooking the nuanced judgment calls that experienced researchers typically provide. Balancing automation with expert oversight is crucial in maintaining data integrity, but the efficiency gained is undoubtedly a compelling reason to embrace this approach.
Integrative Approaches
Combining Techniques
Exploring the combining techniques perspective opens new doors in X-ray diffraction methodologies. By merging single crystal XRD with complementary techniques such as neutron diffraction or electron microscopy, researchers can gather more comprehensive data that furthers understanding of complex systems. The key characteristic is the ability to fill gaps left by individual techniques, thus providing a more holistic view of the material being studied.
An intriguing example comes from materials that exhibit exotic properties, like superconductors. Here, using a combination of XRD and other techniques helps unravel their structural underpinnings and electron interactions, leading to new discoveries. However, managing the intricacies of these combined approaches can be a daunting task, often requiring specialized knowledge and methods to sift through the added complexities of the datasets.
Interdisciplinary Applications
Lastly, the interdisciplinary applications of single crystal X-ray diffraction are gaining momentum. By integrating knowledge from various fields, such as chemistry and biology, new answers to long-standing questions are being uncovered. This approach emphasizes the versatility of XRD in tackling diverse scientific challenges, whether it’s deciphering the crystal structures of novel pharmaceutical compounds or understanding biomolecular assemblies in structural biology.
The unique feature of this trend is its potential for cross-pollination of ideas, where methods and knowledge from one domain can rekindle insights in another. However, the challenges come with aligning different scientific cultures and methodologies, which may lead to conflicting approaches at times. Successfully fostering collaboration among diverse teams is essential for the fruitful application of single crystal X-ray diffraction in broader scientific contexts.
"The advances in single crystal X-ray diffraction are not just incremental. They represent a critical shift towards comprehensive structural understanding across multiple disciplines."
In summary, as we look ahead, the future of single crystal X-ray diffraction appears promising. Technological advancements and empathic interdisciplinary collaborations stand to reshape the landscape, driving forward both practical applications and foundational research.
Epilogue
The conclusion of this article serves as a pivotal section, encapsulating the core findings and implications of single crystal X-ray diffraction. This technique stands at the intersection of various scientific disciplines, particularly in material science, chemistry, and biotechnology. It not only enables researchers to decipher the complex structures of crystalline materials but also plays a critical role in advancing drug design and material development.
Summary of Key Findings
In reviewing the essential aspects covered in the article, several key points emerge:
- Methodological Diversity: We explored various methodologies, from the fundamental principles of X-ray interaction with matter to advanced data processing techniques. Such diversity allows for precise structural analysis across different complexes and materials.
- Applications in Various Fields: The applications of single crystal X-ray diffraction span structural biology, materials science, and pharmaceuticals. It has facilitated breakthroughs in understanding enzyme mechanisms, characterizing new materials, and analyzing drug-receptor interactions.
- Challenges and Limitations: Despite its significance, challenges persist, particularly in the crystallization of suitable samples and ensuring high data quality. These aspects underscore the ongoing need for innovation and technical improvements in the field.
- Future Implications: The article also highlighted technological advancements and integrative approaches that promise to shape the future of single crystal X-ray diffraction. High-resolution techniques and automated data collection are setting the stage for a more streamlined analysis process.
Impact on Scientific Research
The impact of single crystal X-ray diffraction on scientific research cannot be overstated. By providing a detailed view of molecular arrangements at an atomic level, this technique enlightens various research domains:
- Unraveling Molecular Mysteries: In structural biology, the understanding of protein structures through this method has led to profound insights into biological mechanisms. It's like peeling an onion—layer by layer, the structural complexity unfolds, revealing the intricacies of life itself.
- Material Innovations: In materials science, the ability to grasp the atomic arrangement of materials paves the way for engineering new substances with desired properties. Consequently, advancements in fields such as nanotechnology and electronic devices have roots in findings provided by X-ray diffraction studies.
- Driving Pharmaceutical Breakthroughs: The role of X-ray diffraction in drug design and development is monumental. By understanding how drugs interact with their targets at a molecular level, scientists can engineer better therapeutics. It fosters a dynamic approach to designing effective medications that can tackle complex health issues.
In summary, the reflections on the challenges, future trends, and core findings discussed in this article elucidate the crucial role of single crystal X-ray diffraction in bridging gaps in knowledge across scientific disciplines.
Thus, as we chart the course forward in research and development, the principles and applications of single crystal X-ray diffraction will undoubtedly continue to illuminate new paths and possibilities.